Evolution of the gene regulatory network of body axis by enhancer hijacking in amphioxus
Abstract
A central goal of evolutionary developmental biology is to decipher the evolutionary pattern of gene regulatory networks (GRNs) that control embryonic development, and the mechanism underlying GRNs evolution. The Nodal signaling that governs the body axes of deuterostomes exhibits a conserved GRN orchestrated principally by Nodal, Gdf1/3, and Lefty. Here we show that this GRN has been rewired in cephalochordate amphioxus. We found that while the amphioxus Gdf1/3 ortholog exhibited nearly no embryonic expression, its duplicate Gdf1/3-like, linked to Lefty, was zygotically expressed in a similar pattern as Lefty. Consistent with this, while Gdf1/3-like mutants showed defects in axial development, Gdf1/3 mutants did not. Further transgenic analyses showed that the intergenic region between Gdf1/3-like and Lefty could drive reporter gene expression as that of the two genes. These results indicated that Gdf1/3-like has taken over the axial development role of Gdf1/3 in amphioxus, possibly through hijacking Lefty enhancers. We finally demonstrated that, to compensate for the loss of maternal Gdf1/3 expression, Nodal has become an indispensable maternal factor in amphioxus and its maternal mutants caused axial defects as Gdf1/3-like mutants. We therefore demonstrated a case that the evolution of GRNs could be triggered by enhancer hijacking events. This pivotal event has allowed the emergence of a new GRN in extant amphioxus, presumably through a stepwise process. In addition, the co-expression of Gdf1/3-like and Lefty achieved by a shared regulatory region may have provided robustness during body axis formation, which provides a selection-based hypothesis for the phenomena called developmental system drift.
Editor's evaluation
This valuable work advances our understanding of how deuterostome body plan is established during development as well as how the gene regulatory network governing early embryogenesis has been rewired during animal evolution. The evidence supporting the conclusions is compelling, with a suite of molecular-level experiments. The work will be of broad interest to developmental biologists.
https://doi.org/10.7554/eLife.89615.sa0Introduction
The developmental regulatory mechanisms of body axis formation have been investigated in numerous metazoans, which have greatly advanced evolutionary developmental biology (evo-devo) as a research field (Hall, 1999). Importantly, these accumulated data also make it possible to analyze how the gene regulatory networks (GRNs) controlling body axes has been evolving in different clades, a key theme in evo-devo (Peter and Davidson, 2011; Hall, 1999). Nevertheless, detailed functional genetic evidence showing how GRNs could be rewired during evolution is usually lacking, which hinders our understanding of the evolvability of organisms.
Nodal signaling plays a conserved role in patterning the dorsal–ventral (D–V) and left–right (L–R) axes in deuterostomes, exhibiting a conserved GRN orchestrated principally by Nodal, Gdf1/3, and Lefty (Jia and Meng, 2021). Different from most deuterostomes which has one Gdf1/3 gene, mammals and frogs have two such genes (namely Gdf1 and Gdf3), derived from lineage-specific duplications (Opazo and Zavala, 2018; Opazo et al., 2019). For simplicity, we collectively call them Gdf1/3 genes in this study. The expression patterns of genes coding Nodal and Gdf1/3 are highly conserved in echinoderms and vertebrates, with Nodal being expressed zygotically (unilaterally at neurula or larva stage) (Supplementary file 1a), and Gdf1/3 both maternally and zygotically (but bilaterally at neurula stage) (Supplementary file 1b). Zygotic Nodal functions synergistically with preexisting maternal Gdf1/3 by forming heterodimers to activate the signaling pathway (Montague and Schier, 2017; Opazo et al., 2019). Robust Nodal signaling is safeguarded by a positive-feedback loop from further activation of Nodal itself and a negative feedback loop through inducing the expression of Lefty encoding an inhibitor of the signaling (Müller et al., 2012).
Previous studies have identified one Nodal, one Lefty and two Gdf1/3 (tentatively named GDF1/3-like1/Vg1 and GDF1/3-like2) genes in basally divergent chordate amphioxus (Yu et al., 2002; Satou et al., 2008; Onai et al., 2010). The expression pattern of GDF1/3-like2 has not been investigated before this study. Like the orthologs in echinoderms (Duboc et al., 2008) and vertebrates (Meno et al., 1997; Bisgrove et al., 1999; Ishimaru et al., 2000; Tanegashima et al., 2000), Lefty gene in amphioxus is expressed zygotically and unilaterally (Onai et al., 2010; Soukup et al., 2015; Morov et al., 2016; Zhang et al., 2019). Unusually, it was found that Nodal and GDF1/3-like1/Vg1 are both maternally supplied and zygotically expressed unilaterally at the neurula stage (Supplementary file 1a and b). This implies that the regulatory network governing the Nodal signaling pathway has undergone alterations within this particular clade, while its function in D–V and L–R axes patterning has been preserved (Onai et al., 2010; Soukup et al., 2015; Li et al., 2017; Zhang et al., 2019). We thus speculate that the Nodal signaling of amphioxus would be an excellent case to trace the evolutionary history of a GRN and to clarify the mechanism underlying it. To evaluate this, we analyzed the GRN of amphioxus Nodal signaling using mutant and transgenic lines and dissected its evolutionary history by integrating available functional genetic data from other deuterostomes.
Results
Evolution of the two Gdf1/3 genes in amphioxus
Gdf1/3 genes have only been detected in deuterostomes (Stenzel et al., 1994; Lapraz et al., 2006; Satou et al., 2008; Simakov et al., 2015; Opazo and Zavala, 2018). The gene is linked to Bmp2/4 in many deuterostome species (Figure 1A; Range et al., 2007; Satou et al., 2008), though this linkage is lost in higher vertebrates like mice, chickens, and frogs (Opazo and Zavala, 2018). Accordingly, sequence similarity and phylogenetic evidence supported the view that Gdf1/3 originated from Bmp2/4 by a tandem duplication event that occurred in the common ancestor of deuterostomes (Figure 1A, Figure 1—figure supplement 1; Lapraz et al., 2006; Range et al., 2007; Satou et al., 2008). However, Floridae amphioxus (Branchiostoma floridae) was reported to contain two Gdf1/3 genes (Satou et al., 2008), one of which (previously named GDF1/3-like2 by Satou et al., 2008) is linked to Bmp2/4 as was found in other deuterostomes, representing the ancestral amphioxus Gdf1/3 gene (hereafter renamed Gdf1/3, Figure 1A). Intriguingly, the other one (previously known as GDF1/3-like1 [Satou et al., 2008] or Vg1 [Onai et al., 2010]), here renamed as Gdf1/3-like (Figure 1A), is also linked to a transforming growth factor-β (TGF-β) family gene Lefty (Satou et al., 2008). This peculiar gene arrangement, together with that the searches of Lefty ortholog were positive only in deuterostomes until 2008, led Satou et al. proposing that the Gdf1/3-like–Lefty gene pair derived from either a duplication of the Gdf1/3–Bmp2/4 gene pair or two duplications of Gdf1/3 (Gdf1/3 duplicated first to generate Gdf1/3-like, which then translocated and duplicated tandemly to generate Lefty) (Satou et al., 2008). However, this view was not supported by molecular phylogenetic analysis (Satou et al., 2008) and was rejected by the recent finding of Lefty genes in Lophotrochozoa (Heger et al., 2020). Further survey on recently updated genomes of bilaterians, especially those reported to have Lefty genes, revealed that the Gdf1/3-like gene and its linkage to Lefty exist only in amphioxus species, but not in any other bilaterians examined (Figure 1A). These findings suggest that the Gdf1/3-like gene most likely arose in Cephalochordata, or at least in the genus of Brachiostoma, through a tandem duplication of Gdf1/3, followed by a translocation of it to the Lefty locus (Figure 1A). In line with this proposal, lineage-specific duplication of the Gdf1/3 gene and translocation of the duplicate to other genomic regions have also been found in at least two lineages of vertebrates (Opazo and Zavala, 2018; Opazo et al., 2019).
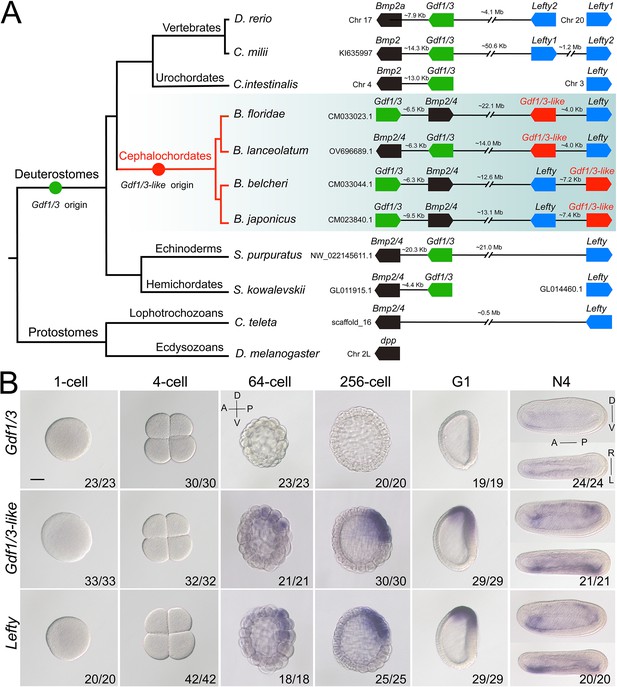
Synteny and expression pattern of amphioxus Gdf1/3 and Gdf1/3-like gene.
(A) Arrangement of Gdf1/3, Bmp2/4, Gdf1/3-like, and Lefty genes in representative bilaterian genomes. Black lines represent the genes (boxes) at both ends are tightly linked and fractured lines represent the genes are close together on a same chromosome or scafford indicated. zebrafish (Danio rerio), elephant shark (Callorhinchus milii), vase tunicate (Ciona intestinalis), Florida amphioxus (B. floridae), European amphioxus (B. lanceolatum), Asia amphioxus (B. belcheri and B. japonicum), sea urchin (Strongylocentrotus purpuratus), acorn worm (Saccoglossus kowalevskii), polychaete worm (Capitella teleta), and fruit fly (Drosophila melanogaster). (B) The spatiotemporal expression pattern of Gdf1/3, Gdf1/3-like, and Lefty at different stages of B. floridae embryos. Embryos at 64-cell to G1 stage were viewed from the left side with anterior to the left (the anterior-posterior [A–P] and D–V axes are labeled), and those at N4 stage were viewed from either the left site (upper panels) or dorsal side (lower panels) with anterior to the left (the A–P, D–V, and L–R axes are all labeled). Numbers in the panels indicate the number of times the expression pattern shown was identified, out of the total number of embryos identified. Scale bar, 50 μm.
Amphioxus Gdf1/3 lost the ancestral role in body axes formation
We next asked if the Gdf1/3 in amphioxus is functionally similar to its ortholog in vertebrates and sea urchins. We first analyzed its embryonic expression pattern using different methods (in situ hybridization, quantitative real-time polymerase chain reaction, and published RNA-seq data; Marlétaz et al., 2018). Unexpectedly, no Gdf1/3 transcripts were detected in amphioxus embryos before the neurula stage, and very weak expression of the gene was found in few cells of the anterior ventral pharyngeal region during the late neurula and larva stages (Figure 1B, Figure 1—figure supplements 2–4). We further generated amphioxus mutants of the Gdf1/3 gene. Consistent with its restricted and weak expression pattern, the homozygous Gdf1/3 mutants (Gdf1/3−/−) displayed normal D–V and L–R axis patterning as the wild-type (WT) by the 3-gill slit stage (Figure 2—figure supplement 1). These data suggested that Gdf1/3 has disassociated from the GRN of body axis formation in living amphioxus. It is, however, notable that overexpression of Gdf1/3 by injecting its mRNA could result in the expansion of anterior and dorsal identity (expressing FoxQ2, Wnt3, Brachyury, and Chordin) at the expense of ventral structures (expressing Evx) (Figure 2—figure supplement 2), as overactivation of the Nodal signaling (Onai et al., 2010; Zhang et al., 2019). Moreover, treatment with SB505124, a selective inhibitor of Alk4/5/7, could ventralize the embryos injected (Figure 2—figure supplement 2). These results demonstrated that the Gdf1/3 still works as a ligand of Nodal signaling, although it is dispensable to the body axis formation of extant amphioxus.
Gdf1/3-like is indispensable for body axes formation in amphioxus
As Gdf1/3 is no longer involved in the body axis formation in amphioxus, while injection of Gdf1/3 and Gdf1/3-like mRNA yielded similar phenotypes (Figure 2—figure supplement 2), we hypothesized that Gdf1/3-like is involved in the body axes formation. To test this, we first reanalyzed the expression pattern of Gdf1/3-like in amphioxus using different methods (in situ hybridization, quantitative real-time polymerase chain reaction, and published RNA-seq data; Marlétaz et al., 2018). Inconsistent with previous study (Onai et al., 2010), no maternal expression of Gdf1/3-like was detected in our analyses (Figure 1B, Figure 1—figure supplements 2–4). Zygotic expression of the gene was first detected in the dorsal blastomeres of the vegetal pole at the 32- to 64-cell stage, and then restricted in the dorsal blastopore lip (Spemann organizer) at the gastrula stage and the left side of the embryo from the early neurula stage (Figure 1B, Figure 1—figure supplement 2). Notably, the expression pattern of Gdf1/3-like is similar (although not identical) to that of Lefty (Figure 1B, Figure 1—figure supplements 2–5).
We then created Gdf1/3-like mutants to see if it is required for amphioxus body axis development. Gdf1/3-like−/− embryos showed severe defects in axis formation. At the G5 stage, the Gdf1/3-like−/− embryos failed to flatten dorsally like the WT embryos (Figure 2—figure supplement 3A), and at subsequent stages they lacked most of the anterior and dorsal structures (Figure 2A, Figure 2—figure supplement 3A). In addition, by the L2 stage, there was a minority of Gdf1/3-like+/− larvae showing no left but two-right phenotype (Figure 2A). We further analyzed the expression patterns of the marker genes for various structures in the mutants. At the gastrulae (G5) stage of Gdf1/3-like−/−, the expression of Gsc in dorsal mesoderm, Chordin and Netrin in dorsal mesoderm and neural ectoderm and SoxB1a in dorsal pan-neural ectoderm disappeared, while that of Evx in ventral domain and Ap2 in epidermal ectoderm expanded dorsally (Figure 2B). At the T0 stage of Gdf1/3-like−/−, the expression of Brachyury in notochord, FoxQ2 in anterior ectoderm, Hex in anterior endoderm, m-actin in somites, Otx in forebrain and anterior pharyngeal endoderm, and Wnt3 in hindbrain and spinal cord were absent (Figure 2—figure supplement 3B). Moreover, in a minority of Gdf1/3-like+/− embryos of the T0–T1 stage, the left-sided expression of Pit in preoral pit and Pou4 in oral primordium disappeared, while the right-sided expression of Nkx2.1 in endostyle and FoxE4 in club-shaped gland became bilaterally symmetrical (Figure 2C). These data collectively indicated that Gdf1/3-like is indispensable for body axes formation in amphioxus and its loss-of-function leads to loss of dorsal, anterior, and left identities.
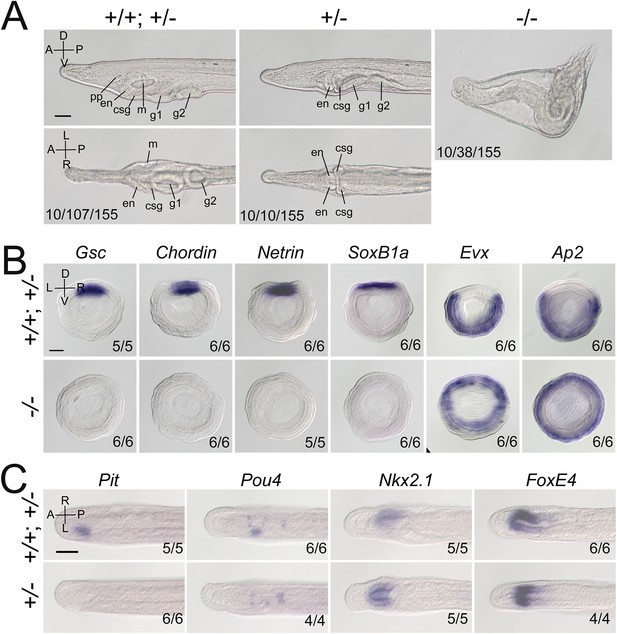
Gdf1/3-like loss-of-function affects amphioxus axes development.
(A) Phenotypic analyses of Gdf1/3-like mutants. Larvae at L2 stage were observed from the left side (upper panels) and ventral side (under panels) with anterior to the left (the A–P, D–V, and L–R axes are labeled). pp, preoral pit; en, endostyle; csg, club-shaped gland; m, mouth; g1, first gill slit; g2, second gill slit. The phenotypes of embryos from a same pool at early stages are provided in Figure 2—figure supplement 3. The three numbers (from left to right) at the bottom right of each panel indicate the number of larvae used for genotyping, the number of larvae with the phenotype, and the total number of larvae examined, respectively. (B, C) The expression of marker genes in Gdf1/3-like mutants. Embryos in (B) were at G5 stage and viewed from the blastopore with dorsal to the top (the D–V and L–R axes are labeled), while embryos in (C) were at T0–T1 stage and viewed from the dorsal side with anterior to the left (the A–P and L–R axes are labeled). Numbers at the bottom right indicate the number of times the genotype shown was identified, out of the total number of examined embryos with the expression pattern. The nomenclature +/+; +/– refer to a pool of animals with different genotypes (+/+ and +/–) in the figures of present study. Scale bars, 50 μm.
In vertebrates, transduction of Nodal signaling requires Gdf1/3 to form heterodimer with Nodal (Montague and Schier, 2017). To see whether Gdf1/3-like is similarly required for Nodal signaling transduction in amphioxus, we examined the expression of Nodal and a target gene (Gsc) of the Nodal signaling in Gdf1/3-like−/− embryos. Previous studies have used Gsc as a Nodal target in both vertebrates (Thisse et al., 1994; Houston and Wylie, 2005; Bisgrove et al., 2017) and invertebrates including amphioxus (Onai et al., 2010; Saudemont et al., 2010; Morov et al., 2016; Zhang et al., 2019). At the G1 stage, the expression of Nodal (most of them are probably maternal) was at a comparable level in Gdf1/3-like+/+, Gdf1/3-like+/−, and Gdf1/3-like−/− embryos (Figure 2—figure supplement 4). However, at the same stage the expression of Gsc was activated in the dorsal blastopore lip of Gdf1/3-like+/+ embryos but disappeared or was reduced in Gdf1/3-like−/− embryos. Interestingly, we also noticed that in minority of Gdf1/3-like+/− embryos, the Gsc expression was reduced compared to Gdf1/3-like+/+ embryos (Figure 2—figure supplement 4). These results suggested that in the absence of Gdf1/3-like, Nodal alone could not activate the Nodal signaling in amphioxus.
Maternal and zygotic Nodal is necessary for amphioxus body axes formation
In amphioxus, Nodal is expressed both maternally and zygotically (Onai et al., 2010). To dissect its function during embryogenesis, we generated Nodal heterozygous animals and crossed them to analyze homozygous mutants. Nodal−/− embryos exhibited defects of L–R axis (Figure 3—figure supplement 1) as Gdf1/3-like+/− (shown above) and embryos in which late Nodal signaling activity was blocked (Soukup et al., 2015; Li et al., 2017). This result showed that zygotic Nodal is necessary for L–R patterning in amphioxus.
However, unlike Gdf1/3-like−/− mutants but like some Gdf1/3-like+/− mutants (Figure 2A), these Nodal−/− mutants do not show obvious dorsal–ventral or anterior–posterior defects (Figure 3—figure supplement 1). This is probably related to maternal Nodal expression. We therefore generated maternal Nodal mutants (MNodal). Since zygotic Nodal mutants could not survive into adulthood, we screened for genetic mosaic females (founders) carrying oocytes of biallelic mutations (Shi, 2022) at the Nodal locus (Figure 3—figure supplement 2A). Two such founders (named founders 1 and 2) were identified from nearly one hundred of animals. In situ hybridization experiment revealed that around 50% and 20% of eggs released by the founders 1 and 2, respectively, showed no maternal Nodal mRNA accumulation (Figure 3A, B). In line with this, when eggs of the two founders were fertilized with WT sperms (Figure 3—figure supplement 2B), around 50% and 20% of them (MNodal) showed a mild ventralized phenotype, respectively (Figure 3C, D, Figure 3—figure supplement 2C). Among embryos generated from the above two crosses, approximately 50% and 20% of them, respectively, showed reduced expression of anterior and dorsal markers (Gsc, Chordin, Netrin, SoxB1a, Brachyury, Wnt3, m-actin, and FoxQ2), and expanded expression of the ventral markers (Evx and Ap2) (Figure 3E–F, Figure 3—figure supplement 3). We also crossed the two founders with male Nodal+/− animals to generate maternal and zygotic Nodal mutants (MZNodal). MZNodal embryos, as expected, accounting for around 25% and 10% of offspring of the founders 1 and 2, respectively, displayed ventralized phenotypes being more severe than MNodal mutants, but comparable to Gdf1/3 mutants (Figure 3C, D, Figure 3—figure supplement 2C). Gene expression analysis showed that MZNodal mutants lost most of the dorsal and anterior identities, as indicated by loss of expressions of the dorsal and anterior marker genes (Gsc, Chordin, Netrin, SoxB1a, Brachyury, Wnt3, m-actin, and FoxQ2), and expanded expressions of the ventral markers (Evx and Ap2) (Figure 3E, F, Figure 3—figure supplement 3). These results collectively indicated that maternal Nodal, probably zygotic Nodal as well, is required for regulating the D–V axis formation in amphioxus.

Nodal gene is required for amphioxus axis development.
(A) Nodal expression in amphioxus eggs from different females (wild-type [WT], founder 1 [F0♀1] and founder 2 [F0♀2]). They were observed with animal pole to the top (circles indicate polar body). Two types of Nodal expression (normal and deficient) were observed. (B) Histogram showing the percentage of eggs of normal or deficient maternal Nodal accumulation from different females. (C) Phenotypic analyses of maternal (MNodal) and maternal–zygotic (MZNodal) Nodal mutants. They were observed from the left side with anterior to the left at N2 stage (the A–P and D–V axes are labeled). Phenotype of the two mutants from a same pool at other stages are provided in Figure 3—figure supplement 2. (D) The percentage of embryos of different phenotypes as shown in (C). Embryos from five different crosses were examined. (E) The expression of marker genes in MNodal and MZNodal mutants at G5 stage. All embryos were viewed from the blastopore with dorsal to the top (the D–V and L–R axes are labeled). (F) The percentage of embryos showing different expression patterns (normal, MNodal, and MZNodal) as indicated in (E). Embryos from three different crosses were examined. Scale bars, 50 μm (A, C, E). The total number of analyzed eggs or embryos are listed under each column (B, D, F).
-
Figure 3—source data 1
The number of eggs or embryos with different phenotypes or expression patterns.
- https://cdn.elifesciences.org/articles/89615/elife-89615-fig3-data1-v1.xlsx
The phenotypes of MZNodal and Nodal−/− are strikingly similar to those of Gdf1/3-like−/− and Gdf1/3-like+/−, respectively, suggesting that Nodal is indispensable for Gdf1/3-like activity during Nodal signal transduction. To further test this, we injected either Nodal or Gdf1/3-like mRNA into maternal MNodal embryos and analyzed the Gsc expression at the G5 stage. We found that Nodal mRNA injection could rescue Gsc expression in the MNodal embryos, while Gdf1/3-like mRNA injection could not, although it expanded the Gsc expression domain in normal embryos (Figure 3—figure supplement 4). This result demonstrated that Gdf1/3-like alone (without Nodal) is unable to activate Nodal signaling in amphioxus.
Gdf1/3-like hijacked the regulatory region of Lefty
As Gdf1/3-like is linked to Lefty in a head-to-head way in all sequenced amphioxus genomes and the two genes exhibit a similar expression pattern during embryogenesis, we hypothesized that the intergenic region between the two genes might include most if not all cis-regulatory elements (such as enhancers) required for their expression. To test this, we cloned the intergenic region (about 4 kb) into a pminiTol2 plasmid carrying a mCherry reporter and generated a stable amphioxus transgenic line carrying it (Figure 4A). Whole-mount in situ hybridization analysis revealed that the transcription of mCherry was similar (although not identical) to that of endogenous Gdf1/3-like and Lefty with simultaneous initiation and D–V and L–R asymmetric expression pattern (Figure 4A). We further made a dual-reporter pminiTol2 construct, in which the coding sequences of eGFP and mCherry were inserted into the two ends of the 4 kb region, respectively, and injected it into amphioxus embryos. In situ analysis of transient transgenic embryos showed that at the G1 stage, the 4 kb sequence could simultaneously drive eGFP and mCherry transcription in a similar pattern in the dorsal organizer region of the injected embryos (Figure 4B). These results indicated that the intergenic sequence indeed contains sequence elements required for regulating the expression of the two linked genes.
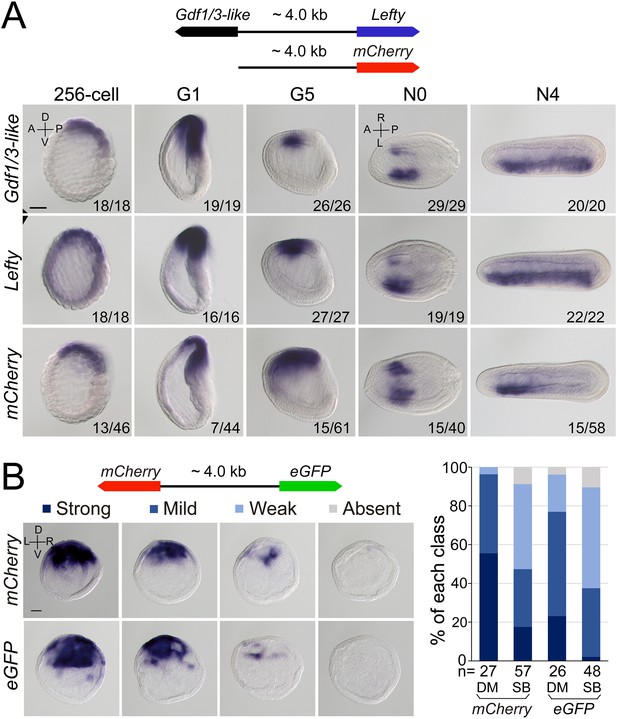
Regulatory activity of the intergenic region between Gdf1/3-like and Lefty genes.
(A) The top schematic diagram shows the 4 kb region between Gdf1/3-like and Lefty and the construct used to generate stable amphioxus transgenic lines. Embryos used for in situ analysis were from a cross between a female founder and a wild-type (WT) male. The bottom panels show the expression patterns of Gdf1/3-like, Lefty, and mCherry reporter in transgenic embryos. Embryos at 256-cell to G5 stages were viewed from the left side (the A–P and D–V axes are labeled), and those at N0 and N4 stages were viewed from the dorsal side (the A–P and L–R axes are labeled). Numbers at the bottom right of each panel indicate the number of times the expression pattern was observed, out of the total number of examined embryos. (B) The left top schematic diagram shows the dual-reporter construct used in this analysis, and the panels below it shows the expression of mCherry and eGFP in embryos injected with the construct and then treated with dimethyl sulfoxide (DM) or SB505124 (SB). All embryos are at G1 stage viewed form the blastopore with dorsal to the top (the D–V and L–R axes are labeled). Four categories of expression (strong, mild, weak, and absent) for both mCherry and eGFP were observed in the dorsal blastopore lip of embryos, and their percentages are shown in the right histogram. The total number of analyzed embryos are listed under each column. Scale bars, 50 μm. The effect of SB505124 treatment on Nodal signaling was validated by the phenotype of larvae treated (shown in Figure 4—figure supplement 2).
-
Figure 4—source data 1
The number of embryos with different expression patterns.
- https://cdn.elifesciences.org/articles/89615/elife-89615-fig4-data1-v1.xlsx
After demonstrating the bidirectional activity of the 4 kb intergenic sequence in driving Lefty and Gdf1/3-like genes, we then asked which one of the two genes originally used this region to regulate its expression. In sea urchin and vertebrate embryos, the expression pattern of Lefty and Gdf1/3 genes are different, with the former being zygotically activated at blastula stage and unilaterally expressed from gastrula/neurula stage (Meno et al., 1996; Meno et al., 1997; Bisgrove et al., 1999; Ishimaru et al., 2000; Tanegashima et al., 2000; Duboc et al., 2005; Duboc et al., 2008), and the latter being maternally preloaded and bilaterally expressed from gastrula/neurula stage (Supplementary file 1b). Moreover, Lefty expression depends on Nodal signaling in these two groups (Saudemont et al., 2010; Bisgrove et al., 2017), while Gdf1/3 expression does not, at least in sea urchin (Range et al., 2007). As demonstrated above, Lefty expression pattern in amphioxus is similar to its orthologs in sea urchin and vertebrates, but Gdf1/3-like expression follows essentially that of Lefty. Additionally, we and others also showed that Lefty expression in amphioxus embryos depends on Nodal signaling (Soukup et al., 2015; Morov et al., 2016; Li et al., 2017; Zhang et al., 2019). These results therefore implied that the 4 kb region was initially responsible for Lefty expression, and then hijacked by Gdf1/3-like after it was translocated to the current locus in amphioxus ancestor. To evaluate this scenario further, we examined Gdf1/3-like expression in the MNodal embryos to see if it is dependent on Nodal signaling as that of Lefty. The result showed that, in MNodal embryos, the expression of Gdf1/3-like and Lefty were both significantly reduced or abolished at the early gastrula stages (G1–G3), although their initial expressions were not affected at the blastula stage (256-cell) and their expressions re-appeared at late gastrula stage (G5) (Figure 4—figure supplement 1). To examine this more directly, we injected amphioxus embryos with the above dual-reporter construct and then treated them with Nodal signaling inhibitor SB505124 to see if the expression patterns of both reporter genes are affected. In situ result showed that compared to untreated embryos, SB505124-treated embryos exhibited decreased expression for both eGFP and mCherry genes (Figure 4B). This indicates that the 4 kb region includes the sequence elements required for Lefty and Gdf1/3-like expression regulation by Nodal signaling. Together, these results imply that the regulatory logic of Lefty expression is conserved in different deuterostomes and the regulatory elements used to regulate Lefty expression was hijacked by Gdf1/3-like after its translocation next to Lefty gene in amphioxus ancestor.
Discussion
During evolution, morphological innovations are usually accompanied by changes of GRNs essential for developmental processes (Peter and Davidson, 2011). However, the GRNs underpinning a trait in a common ancestor could also diverge in descendant lineages even as the trait itself remains conserved, a phenomenon called developmental system drift (True and Haag, 2001; Haag and True, 2021). In either way, functional genetic evidence with a robust phylogenetic framework to demonstrate how GRN changes could have happened is sparse so far, although the underlying mechanism for the evolvability has been usually attributed to changes of cis-regulatory elements (Peter and Davidson, 2011). The most striking discovery in our study is that enhancer hijacking events could trigger the evolution of a GRN. In addition, through detailed analyses of available functional genetic data below, we could demonstrate how a GRN could have evolved in a stepwise way, in the absence of ‘molecular developmental fossils’ (Figure 5).

Scenario for evolution of the Nodal signaling in amphioxus.
The situation in sea urchin (echinoderm) and zebrafish (vertebrate) represents an ancestral scenario which has been rewired and modified in amphioxus through at least three sequential steps as shown in dashed box. D–V, dorsal–ventral axis; L–R, left–right axis; Ab–O, oral–aboral axis. The dorsal–ventral orientation of Chordata was inversed relative to that of Ambulacraria during evolution (Lowe et al., 2015), thus the oral and left side of sea urchin corresponds to the dorsal and right side of zebrafish and amphioxus, respectively.
Nodal signaling plays an essential role for patterning the D–V and L–R axis in deuterostomes (Jia and Meng, 2021). The expression patterns of the genes coding the ligands of the signaling pathway are conserved in extant echinoderms and vertebrates (Supplementary file 1a, b). Thus, the GRN of the Nodal signaling pathway revealed in these species most likely represents an ancestral scenario: Gdf1/3 is preloaded in an egg; by blastula stage, Nodal and Lefty appear zygotically; Nodal functions interdependently with the preexisting Gdf1/3 to activate the signaling pathway, while Lefty restrains the activity of the signaling pathway by inhibiting the signaling (Figure 5; Müller et al., 2012; Montague and Schier, 2017). Our data showed that this highly conserved GRN has been rewired and modified in cephalochordate amphioxus: Nodal is expressed maternally in an egg; ancestral Gdf1/3 lost its expression and function at embryonic stage, while Gdf1/3-like (a duplicate of Gdf1/3) recruited the regulatory elements of Lefty and is expressed in a similar pattern as Lefty from the blastula stage; Gdf1/3-like functions interdependently with the preexisting Nodal to activate the signaling pathway; and Lefty still acts as a feedback inhibitor of the signaling pathway (Figure 5). Our results and those from previous studies in other species enable us to infer that at least three sequential steps are probably required to evolve the GRN as found in extant amphioxus from an ancestral situation as reported in echinoderms and vertebrates (as discussed below): (1) the Gdf1/3-like originated and translocated adjacently to Lefty and hijacked its enhancers; (2) the Gdf1/3 retreated from the Nodal signal; (3) the Nodal became maternally expressed (Figure 5).
Overexpression of Gdf1/3 in zebrafish and Xenopus embryos have no effects on axes development (Dale et al., 1993; Bisgrove et al., 2017). It is therefore expected that the emergence of Gdf1/3-like would not affect the development and viability of the ancestor of extant amphioxus (i.e. step 1, Figure 5). In contrast, disruption of maternal Gdf1/3 function is lethal in sea urchin and zebrafish embryos (Range et al., 2007; Bisgrove et al., 2017; Montague and Schier, 2017; Pelliccia et al., 2017). This indicates that the retreatment of the Gdf1/3 ortholog from the Nodal signaling (step 2) could not happen before the emergence of Gdf1/3-like and its recruitment of the Lefty enhancers (step 1). Similarly, overexpression of Nodal in the presence of maternal Gdf1/3 led to defects in axis formation of zebrafish embryos (Bisgrove et al., 2017), suggesting that Nodal could not become a maternal factor before the retreatment of Gdf1/3 (i.e. step 2 happened before step 3). These suggested that the 1–2–3 ordered events could have led to the situation in extant amphioxus (Figure 5). Injection of Gdf1/3 mRNA to the yolk syncytial layer, where Nodal is expressed endogenously, was sufficient to rescue MGdf1/3 defects in zebrafish, thus the ubiquitous distribution of maternal Gdf1/3, in the region where Nodal is not expressed, is dispensable (Montague and Schier, 2017). Once the Gdf1/3-like started its current expression pattern by hijacking Lefty enhancers, the function of Gdf1/3-like and Gdf1/3 became redundant, allowing the retreatment of Gdf1/3 from the signaling pathway (i.e. step 2, Figure 5). As discussed in the study of zebrafish (Montague and Schier, 2017), preloading of a maternal factor (Gdf1/3 in zebrafish or Nodal in amphioxus) could be instrumental for ensuring Nodal signaling initiation in a rapid and temporally reliable manner. Likewise, the establishment of Nodal as a maternal factor may be a compensation mechanism for the retreatment of ubiquitously maternal Gdf1/3 (i.e. step 3, Figure 5). Zygotic Nodal mutants failed to form L–R axis but formed D–V axis normally in amphioxus (Figure 3—figure supplement 1), suggesting that maternal Nodal is redundant to compensate the D–V axis formation. However, maternal Nodal mutants formed only partial anterior and dorsal structures (Figure 3, Figure 3—figure supplements 2 and 3), suggesting that the expression of zygotic Nodal might be delayed in living amphioxus, compared to that of the amphioxus ancestor before step 3. Our proposed scenario highlights that enhancer hijacking by Gdf1/3-like was a triggering and probably ‘neutral’ step for the rewiring of the body axes GRN in amphioxus ancestors.
Although developmental system drift is probably universal in long evolutionary periods (Palmer, 2004), it has been rarely possible to analyze whether there is selective advantage of such drifts. Co-expressed gene pairs sharing intergenic enhancers have been reported in various cases (Ueda et al., 2006; Sumiyama and Tanave, 2020; Zinani et al., 2021; Zinani et al., 2022), and often fall into the same functional categories (Zinani et al., 2022). In zebrafish, the paired her1 and her7 can provide robustness for segmentation patterning (Zinani et al., 2021). The two proteins need to form dimers to inhibit their own transcription, thus forming a negative-feedback loop to maintain a stable level of both proteins in cells (Zinani et al., 2021). In our case, Gdf1/3-like functions as an activator of the signaling that maintains the transcriptions of both Gdf1/3-like and Lefty in a positive-feedback loop, while Lefty acts as a repressor of the signaling forming a negative-feedback loop (Li et al., 2017; Zhang et al., 2019). The co-expression of Gdf1/3-like and Lefty gene pair (Figure 1B, Figure 1—figure supplements 2–5) achieved by sharing regulatory region (Figure 4) likely safeguards a dosage balance between the positive- and negative-feedback loop. This is likely an advantage for robust pattern formation as suggested in other gene pairing systems (Zinani et al., 2022).
Gdf1/3 is one of the reported examples of small-scale gene duplications in amphioxus (Brasó-Vives et al., 2022), anurans, and mammals (Opazo and Zavala, 2018). However, these genes appear to have evolved differently: in anurans and mammals both Gdf1/3 paralogs retain the ancestral function in axis formation (Birsoy et al., 2006; Andersson et al., 2007; Vonica and Brivanlou, 2007), while in amphioxus only Gdf1/3-like undertakes this function but Gdf1/3 seems to have lost its original function. As discussed above, hijacking of Lefty enhancers by Gdf1/3-like had enabled Gdf1/3-like to be functionally redundant to Gdf1/3, allowing Gdf1/3 to retrieve from its ancestral function in regulating body axis formation through changing its expression pattern. In addition, the redundancy of Gdf1/3-like appears to have also relaxed the selection pressure on Gdf1/3-coding region, since Gdf1/3-like mRNA is more efficient in inducing anterior and dorsal expansion than that of Gdf1/3 (Figure 2—figure supplement 2).
We have demonstrated that functional genetic data could be used to dissect the route of GRNs evolution, once there is available data from different species for comparisons. Our study represents an example that illustrated how the GRNs could have shifted in a stepwise way without altering conserved phenotypes, such as body axes that has persisted at least since the Early Cambrian period (Martindale, 2005; Dunn et al., 2014). Our case also showed that enhancer hijacking could be a mechanism underlying the evolution of developmental GRNs, in addition to the changes of cis-regulatory elements.
Methods
Animals and embryos
WT amphioxus (B. floridae) was obtained from Jr-Kai Yu’s lab and bred in the aquaculture system as reported previously (Li et al., 2012). Mature individuals were induced to lay eggs and release sperm by thermal shock (Li et al., 2013). The developmental stages of amphioxus embryos were defined as recently described (Carvalho et al., 2021).
Gene structures and synteny
Positions of Lefty, Gdf1/3-like, Bmp2/4, and Gdf1/3 genes in the genomes of B. floridae, B. belcheri, B. japonicum, and B. lanceolatum were determined according to two recent studies (Brasó-Vives et al., 2022; Huang et al., 2023), while positions of these genes in sea urchin (S. purpuratus) were retrieved directly from Echinobase, in other species including zebrafish (D. rerio), elephant shark (C. milii), vase tunicate (C. intestinalis), acorn worm (S. kowalevskii), polychaete worm (C. teleta), and fruit fly (D. melanogaster) were retrieved directly from Ensembl database.
Phylogenetic analyses
Amino acid sequences of Gdf1/3 and related TGF-β factors were aligned by ClustalX integrated in MEGA5 software (Tamura et al., 2011). The aligned sequences were then used to construct a phylogenetic tree using the maximum likelihood method with PhyML (http://atgc.lirmm.fr/phyml/; Guindon et al., 2010). The substitution model was automatically selected by Smart Model Selection in PhyML (Lefort et al., 2017).
Quantitative real-time polymerase chain reaction
Embryos or eggs (about 200 per sample) were harvested at desired stages and used to extract total RNA by TRIzol reagent (Ambion). cDNA was synthesized using HiScript III RT SuperMix (+gDNA wiper) kit (Vazyme). Quantitative real-time polymerase chain reaction analysis was performed with ChamQ Universal SYBR qPCR Master Mix kit (Vazyme) on a CFX96 Touch Real-Time PCR Detection System (Bio-Rad) under the conditions of 95°C for 2 min, 40 cycles at 95°C for 5 s, 60°C for 30 s. Expression levels of Nodal, Gdf1/3, Gdf1/3-like, and Lefty were normalized to that of Gapdh (glyceraldehyde-3-phosphate dehydrogenase). Graphs were finished with GraphPad Prism 9 software. Primers for quantitative real-time polymerase chain reaction analysis and their sequences were as follows: BfGdf1/3-like-RT-F (5′-CAAGGGCAAATATCACGACA-3′), Bf Gdf1/3-like-RT-R (5′-TTCACGTCGTCTCTGTCGAA-3′), BfNodal-RT-F (5′-GGACAGACCTCAACGTCACCC-3′), BfNodal-RT-R (5′-CTGAAGACACGCACGGAAAGT-3′), BfLefty-RT-F (5′-CACTGACGCCAGTGGTGCA-3′), BfLefty-RT-R (5′-CGTTGTTGAAAGACTTTCGAGT-3′), BfGdf1/3-RT-F (5′-TTCTCGGCTTTCGTGAACGG-3′), BfGdf1/3-RT-R (5′-ACAGTCCAACCATTTTCGGCA-3′), Gapdh-RT-F (5′-GGTGGAAAGGTCCTGCTCTC-3′), and Gapdh--RT-R (5′-CTGGATGAAAGGGTCGTTAATGG-3′).
Overexpression experiments
Coding sequences of Nodal and Gdf1/3-like were amplified from cDNA templates of amphioxus embryos and ligated into the pXT7 vector using T4 DNA ligase (Promega). Due to low expression level, coding exons of Gdf1/3 were individually amplified from genomic DNA templates and then assembled into the pXT7 vector using a Gibson cloning kit (New England Biolabs). Nodal, Gdf1/3, and Gdf1/3-like mRNA were synthesized using T7 mMESSAGE mMACHINE kit (Ambion). Unfertilized eggs were injected with mRNAs and fertilized as previously described (Liu et al., 2013). The injected embryos were treated, with 0.2% dimethyl sulfoxide (as control) or 50 μM SB505124, from 4-cell stage to G1 stage and were fixed at G4 or N3 stage for whole-mount in situ hybridization.
Generation of mutant animals and embryos
Gdf1/3 mutant were generated using CRISPR/Cas9 system targeting a site (Gdf1/3-sgRNA: 5′-GGCCCGCTGTAGCGATGA-3′) in the first exon. The process of generating founders of Gdf1/3 gene knock-out was implemented as our previous study (Su et al., 2020). F1 Gdf1/3+/− carrying −25 bp mutation were intercrossed to generate Gdf1/3−/− (Figure 2—figure supplement 1). A pair of primer (Gdf1/3-Cas9-F1: 5′-TACCACACATCACCCGGACT-3′/Gdf1/3-PCR-R1: 5′-CACATCCTCGTCTTCCGGTC-3′) and SfcI enzyme were used for genotyping and mutation type analysis.
Gdf1/3-like mutants were generated with a TALEN pair (Gdf1/3-like-Fw: 5′-TTCGACAGAGACGAC-3′/Gdf1/3-like-Rv: 5′-TGCACGGCGCTCACGA-3′) targeting the coding region of the second exon as previously reported (Li et al., 2014; Li et al., 2017). F1 heterozygotes carrying a one base pair insertion (+1 bp) were used and intercrossed to generate homozygote mutants (Gdf1/3-like−/−) (Figure 2—figure supplement 3A). Embryos or tiny tips of adult tail were lysed with Animal Tissue Direct PCR Kit (Foregene) to release the genomic DNA. Genomic region spanning the target site were amplified with primer Gdf1/3-like-TALEN-F: 5′-CGTGACGTACTCCGTGTCTG-3′/Gdf1/3-like-TALEN-R1: 5′-GCTGAAGTGTGGGCAAGAGT-3′ or Gdf1/3-like-TALEN-R0: 5′-CCGTTTGCAGATGTTGCCG-3′. The amplicons were digested with StuI to test the mutations and sequenced to recognize the mutation types.
Nodal heterozygotes (Nodal+/−) were generated using a TALEN pair (Nodal-Fw2/Rv2) in previous study (Shi et al., 2020). Male and female Nodal+/− carrying identical mutation (-7 bp) were intercrossed to generate Nodal−/− (Figure 3—figure supplement 1A). Genotyping for Nodal mutants were implemented as previous study (Shi et al., 2020). A TALEN pair (Nodal-Fw2/Rv2) assembled previously (Shi et al., 2020) and a sgRNA (Nodal-sgRNA:5′-GGCGGAGAGGGTCTGACGCT-3′) reported previously (Su et al., 2020) were used to generate hundreds of chimeric female founders. By adulthood stage, among nearly 100 of animals, an individual generated with Nodal-Fw2/Rv2 (named founder 1, F0♀1) and another individual generated with Nodal-sgRNA (named founder 2, F0♀2) laid eggs lacking maternal Nodal accumulation (Figure 3—figure supplement 2A) with 50% and 20% ratio, respectively. Each female founder was crossed with a male WT, respectively, to generated maternal Nodal mutants (MNodal) and crossed with a male Nodal+/−, respectively, to generated maternal–zygotic Nodal mutants (MZNodal) (Figure 3—figure supplement 2B).
Generation of transgenic animals and embryos
The intergenic region between Gdf1/3-like and Lefty was cloned into the pmini-Chordin-mCherry (Shi et al., 2018) by replacing its Chordin promoter with the intergenic region. The generated pmini-Lefty-mCherry construct was used to generate Lefty::mCherry transgenic amphioxus with method reported previously (Shi et al., 2018). The intergenic region, the coding sequence of mCherry and eGFP sequence were linked into the pminiTol2 plasmid with a Gibson cloning kit (New England Biolabs) to generate pmini-eGFP-Lefty-Gdf1/3-like-mCherry construct. The later was injected into amphioxus embryos. The injected embryos were treated, with 0.2% dimethyl sulfoxide (as control) or 50 μM SB505124, from 4-cell stage to G1 stage.
Whole-mount in situ hybridization and imaging
The RNA probes used in present study were prepared previously (Li et al., 2017; Shi et al., 2018; Zhang et al., 2019), except the probe of eGFP and Gdf1/3 gene, which was prepared using the same method as described in a previous study (Li et al., 2017). Embryos at desired developmental stages were fixed with 4% paraformaldehyde in MOPS buffer (PFA–MOPS, wt/vol) and then stored in 80% ethanol in H2O (vol/vol) at −20°C until needed. Whole-mount in situ hybridization was performed as previously described (Yu and Holland, 2009). After staining, the embryos were mounted in 80% glycerol (vol/vol) for photographing under an inverted microscope (Olympus IX71). Living amphioxus embryos or larvae were observed using the same microscope. We use Photoshop software to automatically stitch together photos of different parts of the embryo or larva, when a single field of view cannot display the whole mount of embryo or larva.
The double in situ hybridization of Lefty and Gdf1/3-like was achieved by using amplification-based single-molecule in situ hybridization (asmFISH) method as described in a previous study (Lin et al., 2021), which hereby was performed using SEERNA ISH RNA Fluorescence in Situ Detection Kit (SEERNA Bioscience, Xiamen, China, Cat# SP1001, SP1002, and SP1003) under the guidance of standard protocol of the manual. The details are as follows. The label probes of Lefty and Gdf1/3-like were conjugated to Cy5 and Alexa fluor 488, respectively (provided in the Kit). For asmFISH, embryos at desired developmental stages were fixed in 4% PFA–MOPS (wt/vol) and then stored in methanol at −20°C until needed. Before hybridization, the embryos were permeabilized in 0.1 M HCl containing 0.1 mg/ml pepsin (Sigma, Cat# P7012) at 37°C (for 5 min at 256-cell and G1 stage, 9 min at G4 stage, 30 min at N2 stage) and washed with Wash Buffer (provided in the Kit). The embryos were then incubated in order with the following solutions: target probes in hybridization mix at 46°C for 4 hr, ligation mix at 37°C for 30 min, splint primers in the circularization mix at 37°C for 30 min, amplification mix for rolling circle amplification at 30°C overnight, label probes in hybridization buffer at room temperature for 30 min. Embryos were washed with the Wash Buffer three times after each step. Finally, the embryos were placed in a Mounting medium (Solarbio, Cat# S2100) containing 0.5 μg/ml 4,6-diamidino-2-phenylindole (Sigma, Cat# D8417), which were then ready for image acquisition using the Zeiss LSM980 microscope.
Data availability
All data generated or analysed during this study are included in the manuscript and supporting file. Source data files containing the numerical data used to generate the figures have been provided.
-
NCBI Gene Expression OmnibusID GSE106430. Functional genomic and transcriptomic analysis of amphioxus and the origin of vertebrate genomic traits [RNA-Seq].
-
NCBI BioProjectID PRJNA603158. Branchiostoma floridae x Branchiostoma belcheri isolate: bbbf Genome sequencing and assembly.
-
NCBI Sequence Read ArchiveID PRJNA603159. Branchiostoma floridae x Branchiostoma belcheri isolate: bbbf Genome sequencing and assembly.
-
NCBI TaxonomyID PRJNA647830. Branchiostoma floridae x Branchiostoma japonicum.
-
NCBI BioProjectID PRJNA602496. Genome sequencing and assembly of three amphioxuses.
-
European Nucleotide ArchiveID PRJEB49647. Branchiosotma lanceolatum reference genome and gene annotation.
References
-
An updated staging system for cephalochordate development: One table suits them allFrontiers in Cell and Developmental Biology 9:668006.https://doi.org/10.3389/fcell.2021.668006
-
Animal phylogeny and its evolutionary implicationsAnnual Review of Ecology, Evolution, and Systematics 45:371–395.https://doi.org/10.1146/annurev-ecolsys-120213-091627
-
Developmental system driftEvolutionary Developmental Biology pp. 99–110.https://doi.org/10.1007/978-3-319-32979-6
-
Asymmetric expression of antivin/lefty1 in the early chick embryoMechanisms of Development 90:115–118.https://doi.org/10.1016/s0925-4773(99)00232-4
-
RTK and TGF-beta signaling pathways genes in the sea urchin genomeDevelopmental Biology 300:132–152.https://doi.org/10.1016/j.ydbio.2006.08.048
-
SMS: Smart Model Selection in PhyMLMolecular Biology and Evolution 34:2422–2424.https://doi.org/10.1093/molbev/msx149
-
Mutagenesis at specific genomic loci of amphioxus Branchiostoma belcheri using TALEN methodJournal of Genetics and Genomics = Yi Chuan Xue Bao 41:215–219.https://doi.org/10.1016/j.jgg.2014.02.003
-
An efficient microinjection method for unfertilized eggs of Asian amphioxus Branchiostoma belcheriDevelopment Genes and Evolution 223:269–278.https://doi.org/10.1007/s00427-013-0441-0
-
The evolution of metazoan axial propertiesNature Reviews. Genetics 6:917–927.https://doi.org/10.1038/nrg1725
-
Regulatory genes in the ancestral chordate genomesDevelopment Genes and Evolution 218:715–721.https://doi.org/10.1007/s00427-008-0219-y
-
Generation of two transgenic amphioxus lines using the Tol2 transposon systemJournal of Genetics and Genomics = Yi Chuan Xue Bao 45:513–516.https://doi.org/10.1016/j.jgg.2018.06.002
-
The regulatory landscape of the Dlx gene system in branchial arches: Shared characteristics among Dlx bigene clusters and evolutionDevelopment, Growth & Differentiation 62:355–362.https://doi.org/10.1111/dgd.12671
-
MEGA5: molecular evolutionary genetics analysis using maximum likelihood, evolutionary distance, and maximum parsimony methodsMolecular Biology and Evolution 28:2731–2739.https://doi.org/10.1093/molbev/msr121
-
Developmental system drift and flexibility in evolutionary trajectoriesEvolution & Development 3:109–119.https://doi.org/10.1046/j.1525-142x.2001.003002109.x
-
Amphioxus whole-mount in situ hybridizationCold Spring Harbor Protocols 2009:pdb.prot5286.https://doi.org/10.1101/pdb.prot5286
Article and author information
Author details
Funding
National Natural Science Foundation of China (32070458)
- Guang Li
National Natural Science Foundation of China (31872186)
- Guang Li
National Natural Science Foundation of China (32070815)
- Yiquan Wang
National Natural Science Foundation of China (32061160471)
- Guang Li
Youth Innovation Foundation of Xiamen (3502Z20206032)
- Guang Li
Xiamen University (start-up fund of Xiamen University)
- Qingming Qu
The funders had no role in study design, data collection, and interpretation, or the decision to submit the work for publication.
Acknowledgements
We thank all team members of the GL and QQ laboratories, and Prof. Zhenghong Zuo for helpful discussions. This work is supported by grants from the National Natural Science Foundation of China (nos. 32070458, 31872186, 32070815, and 32061160471), the Youth Innovation Fund Project of Xiamen (3502Z20206032) and the start-up fund of Xiamen University.
Version history
- Received: May 23, 2023
- Preprint posted: June 11, 2023 (view preprint)
- Accepted: December 19, 2023
- Version of Record published: January 17, 2024 (version 1)
Copyright
© 2024, Shi et al.
This article is distributed under the terms of the Creative Commons Attribution License, which permits unrestricted use and redistribution provided that the original author and source are credited.
Metrics
-
- 403
- views
-
- 78
- downloads
-
- 0
- citations
Views, downloads and citations are aggregated across all versions of this paper published by eLife.
Download links
Downloads (link to download the article as PDF)
Open citations (links to open the citations from this article in various online reference manager services)
Cite this article (links to download the citations from this article in formats compatible with various reference manager tools)
Further reading
-
- Computational and Systems Biology
- Evolutionary Biology
A comprehensive census of McrBC systems, among the most common forms of prokaryotic Type IV restriction systems, followed by phylogenetic analysis, reveals their enormous abundance in diverse prokaryotes and a plethora of genomic associations. We focus on a previously uncharacterized branch, which we denote coiled-coil nuclease tandems (CoCoNuTs) for their salient features: the presence of extensive coiled-coil structures and tandem nucleases. The CoCoNuTs alone show extraordinary variety, with three distinct types and multiple subtypes. All CoCoNuTs contain domains predicted to interact with translation system components, such as OB-folds resembling the SmpB protein that binds bacterial transfer-messenger RNA (tmRNA), YTH-like domains that might recognize methylated tmRNA, tRNA, or rRNA, and RNA-binding Hsp70 chaperone homologs, along with RNases, such as HEPN domains, all suggesting that the CoCoNuTs target RNA. Many CoCoNuTs might additionally target DNA, via McrC nuclease homologs. Additional restriction systems, such as Type I RM, BREX, and Druantia Type III, are frequently encoded in the same predicted superoperons. In many of these superoperons, CoCoNuTs are likely regulated by cyclic nucleotides, possibly, RNA fragments with cyclic termini, that bind associated CARF (CRISPR-Associated Rossmann Fold) domains. We hypothesize that the CoCoNuTs, together with the ancillary restriction factors, employ an echeloned defense strategy analogous to that of Type III CRISPR-Cas systems, in which an immune response eliminating virus DNA and/or RNA is launched first, but then, if it fails, an abortive infection response leading to PCD/dormancy via host RNA cleavage takes over.
-
- Evolutionary Biology
- Neuroscience
Neuropeptides are ancient signaling molecules in animals but only few peptide receptors are known outside bilaterians. Cnidarians possess a large number of G protein-coupled receptors (GPCRs) – the most common receptors of bilaterian neuropeptides – but most of these remain orphan with no known ligands. We searched for neuropeptides in the sea anemone Nematostella vectensis and created a library of 64 peptides derived from 33 precursors. In a large-scale pharmacological screen with these peptides and 161 N. vectensis GPCRs, we identified 31 receptors specifically activated by 1 to 3 of 14 peptides. Mapping GPCR and neuropeptide expression to single-cell sequencing data revealed how cnidarian tissues are extensively connected by multilayer peptidergic networks. Phylogenetic analysis identified no direct orthology to bilaterian peptidergic systems and supports the independent expansion of neuropeptide signaling in cnidarians from a few ancestral peptide-receptor pairs.