Depletion of SMN protein in mesenchymal progenitors impairs the development of bone and neuromuscular junction in spinal muscular atrophy
Abstract
Spinal muscular atrophy (SMA) is a neuromuscular disorder characterized by the deficiency of the survival motor neuron (SMN) protein, which leads to motor neuron dysfunction and muscle atrophy. In addition to the requirement for SMN in motor neurons, recent studies suggest that SMN deficiency in peripheral tissues plays a key role in the pathogenesis of SMA. Using limb mesenchymal progenitor cell (MPC)-specific SMN-depleted mouse models, we reveal that SMN reduction in limb MPCs causes defects in the development of bone and neuromuscular junction (NMJ). Specifically, these mice exhibited impaired growth plate homeostasis and reduced insulin-like growth factor (IGF) signaling from chondrocytes, rather than from the liver. Furthermore, the reduction of SMN in fibro-adipogenic progenitors (FAPs) resulted in abnormal NMJ maturation, altered release of neurotransmitters, and NMJ morphological defects. Transplantation of healthy FAPs rescued the morphological deterioration. Our findings highlight the significance of mesenchymal SMN in neuromusculoskeletal pathogenesis of SMA and provide insights into potential therapeutic strategies targeting mesenchymal cells for the treatment of SMA.
eLife assessment
This important work by Hann et al. advances our understanding of the role of the survival motor neuron (SMN) protein in coordinating pathogenesis of the spinal muscular atrophy (SMA). The authors addressed many concerns raised by the reviewers, providing convincing evidence in terms of skeletal analyses not being able to satisfactorily elucidate SMN regulation of bone development.
https://doi.org/10.7554/eLife.92731.3.sa0Introduction
The survival motor neuron (SMN) protein is a crucial component of the spliceosome complex and is essential for the proper function of all cell types (Mercuri et al., 2022). Deficiency in SMN protein disrupts the formation of spliceosome complexes, ultimately causing splicing defects in multiple genes. Mutations in the SMN1 gene, which encodes the SMN protein, give rise to the neuromuscular disorder spinal muscular atrophy (SMA). SMA is characterized by neuromuscular junctions (NMJs) disruption, muscular atrophy, and alpha motor neuron loss (Mercuri et al., 2022; Burghes and Beattie, 2009). The severity of the disease in humans correlates with the copy number of SMN2, which is a paralog of SMN1 in humans. SMN2 primarily produces less functional exon 7-deleted SMN protein and rarely generates a limited quantity of functional full-length SMN protein via alternative splicing. SMA patients are classified into types 0 through 4 based on the severity and timing of disease onset, predominantly determined by the number of copies of the SMN2 gene they possess. More than 50% of SMA patients are categorized as type 1, characterized by muscle defects with proximal muscle atrophy during infancy, eventually resulting in death within a few years (Mercuri et al., 2022).
Previous studies suggest that the onset of SMA is mainly attributed to SMN loss in motor neurons (Monani et al., 2000; Burghes and Beattie, 2009). Nevertheless, motor neuron-specific SMN deficiency in the SMA mouse model exhibits relatively mild phenotypes compared to whole-body SMA mouse models (Park et al., 2010; McGovern et al., 2015). Furthermore, restoring SMN to motor neurons in SMA mouse models result in only partial rescue in lifespan and neuromuscular defects (Passini et al., 2010; Martinez et al., 2012; McGovern et al., 2015; Besse et al., 2020). Systemic administration of antisense oligonucleotide (ASO), which corrects SMN2 splicing to restore SMN expression, significantly prolongs survival compared to central nervous system (CNS) administration (Hua et al., 2011). In the mouse treated with a systemically delivered ASO, blocking the effect of ASO in the CNS by a complementary decoy did not have any detrimental effect on survival, motor function, or NMJ integrity (Hua et al., 2015). These studies suggest that peripheral SMN plays a crucial part in SMA pathology. Overall, investigating the impacts of SMN depletion in peripheral tissues is critical for alleviating neuromuscular impairments and increasing life expectancy in SMA.
In SMA patients, bone growth retardation has been observed (De Amicis et al., 2021; Kipoğlu et al., 2019; Hensel et al., 2020). Studies using whole-body SMA mouse models have revealed that this is caused by diminished growth plate chondrocyte density and endochondral ossification defects, independent of muscle atrophy (Hensel et al., 2020). However, it is still unclear whether these defects result from the SMN ablation in bone-forming cells, or from a decline in liver-derived insulin-like growth factor (IGF) in SMA patients and mice (Hua et al., 2011; Yesbek Kaymaz et al., 2016). In severe SMA mice, the serum levels of IGF decreased by approximately 60% or became undetected (Hua et al., 2011; Murdocca et al., 2012). The decreased serum IGF levels were attributed to decreased expression of liver genes, including Igf1, IGF binding, and ternary complex protein gene Igfals and Igfbp3. The previous studies suggest that the liver is the primary origin of systemic IGF, as demonstrated by the liver-specific deletion of Igf1 and the knockout of Igfals, which is mostly expressed in the liver (Yakar et al., 1999; Yakar et al., 2002). The double KO mice exhibited a 90% decrease in serum IGF levels and displayed a phenotype of shortened femur length and growth plates. It is thus possible that the decrease in serum IGF levels, resulting from reduced liver IGF pathway genes in SMA mice, has also played a role in the observed bone growth defect.
Mesenchymal progenitor cells (MPCs) derived from the lateral plate mesoderm (LPM) differentiate into various types of limb mesenchymal cells, including bone, cartilage, and intramuscular mesenchymal cells like fibro-adipogenic progenitors (FAPs) (Nassari et al., 2017). Recent studies have revealed the role of FAPs in skeletal muscle homeostasis, as reducing the number of FAPs resulted in diminished muscle regeneration capacity, long-term muscle atrophy, and NMJ denervation (Wosczyna et al., 2019; Uezumi et al., 2021). Our latest research demonstrated that FAP-specific deficiency of Bap1, one of the deubiquitinases, leads to NMJ defects (Kim et al., 2022). These recent findings raise the possibility that FAPs may have a specific role in the pathogenesis of neuromuscular diseases such as SMA. However, it has not been studied if depletion of SMN in FAPs can lead to SMA-like neuromuscular pathology.
In this study, we crossed a limb MPC-specific Cre mouse with a floxed Smn1 exon 7 mouse carrying multiple copies of the human SMN2 gene, allowing us to examine the impact of mesenchymal SMN reduction on SMA pathogenesis. As a result, the mutant mice showed skeletal growth abnormalities and local IGF signaling defects in the growth plate. In addition, our findings indicate that the SMN reduction in FAPs, similar to the extent of severe SMA, causes altered NMJ development.
Results
Bone growth restriction and growth plate defects caused by MPC-specific SMN depletion
To investigate the effects of SMN reduction within MPCs in SMA pathogenesis, we crossed Smn1f7/f7 mice, which possess loxP sites flanking exon 7 of the Smn1 gene (Frugier et al., 2000), with Prrx1Cre mice. This produced Smn1ΔMPC mice (Prrx1Cre; Smn1f7/f7) that lacked the Smn1 gene specifically in Prrx1Cre-expressed limb MPCs that give rise to bone, cartilage, and FAPs (Logan et al., 2002; Leinroth et al., 2022). To ascertain whether mutant mice carrying the SMN2 gene, like SMA patients, present pathological phenotypes, we additionally generated SMN2 2-copy Smn1ΔMPC (Prrx1Cre; Smn1f7/f7; SMN2+/+) and SMN2 1-copy Smn1ΔMPC (Prrx1Cre; Smn1f7/f7; SMN2+/0) mice. Control littermates that lacked Prrx1Cre were used as controls for comparison.
SMN2 0-copy Smn1ΔMPC mice died within 24 hr after birth. Regions where limbs should have formed at E18.5 only had rudimentary limb structures (Figure 1—figure supplement 1A). Furthermore, since the upper head bone did not cover the brain, it was directly attached to the skin and protruded. These observations can be attributed to Prrx1Cre-mediated SMN deletion in the LPM-derived limb MPCs and the craniofacial mesenchyme, which is accountable for the formation of calvarial bone (Wilk et al., 2017). To investigate whether the lack of SMN proteins in MPCs is responsible for bone development abnormalities, we performed alcian blue and alizarin red staining on E18.5 SMN2 0-copy Smn1ΔMPC mutants to analyze the structure of bones and cartilage. The appendages displayed restricted bone and cartilage formations, with scarcely discernible femur and tibia (Figure 1—figure supplement 1B, C). In the cranial region, there was an absence of both cartilage and bone at the location of the calvarial bone, with the parietal bone entirely missing and partially absent frontal bone (Figure 1—figure supplement 1D). Additionally, the sternum, which is one of the bones originating from the LPM (Sheng, 2015), was shorter than the control (Figure 1—figure supplement 1E).
The SMN2 2-copy Smn1ΔMPC mice, carrying two homologous SMN2 genes, did not show any discernible differences from the Prrx1Cre-negative control littermates into adulthood. However, the SMN2 1-copy Smn1ΔMPC mice exhibited reduced body size and shorter limb length compared to the SMN2 1-copy control (Smn1f7/f7; SMN2+/0). To assess postnatal bone growth defects observed in SMA patients and mouse models, we conducted micro-computed tomography (micro-CT) analysis on femurs obtained from postnatal day 14 (P14) SMN2 1-copy Smn1ΔMPC and SMN2 2-copy Smn1ΔMPC mice (Figure 1A). The 3D reconstruction image showed that the SMN2 1-copy mutant femur was smaller than the WT control and SMN2 2-copy mutant, and secondary ossification center is denied. The longitudinal virtual section view displayed reduced trabecular bone in the SMN2 1-copy mutant femur. CT analysis data showed that SMN2 1-copy mutants exhibited reduced femur diaphysis length, diameter, and trabecular bone volume compared to the control group, indicating growth plate-dependent endochondral ossification defects (Figure 1B–D). We then examined femoral bone thickness and diaphyseal bone mineral density (BMD) to determine whether mineralization was normal after bone formation. The thickness of the bone in the mutants did not differ significantly from the control, suggesting that bone mineralization was intact (Figure 1E and Figure 1—figure supplement 2A). Unexpectedly, BMD slightly increased in SMN2 1-copy mutants compared to the control group (Figure 1—figure supplement 2B). To assess the impact of osteoclasts and osteoblasts on diaphysis cortical bone mineralization, we utilized Itgb3 immunofluorescence as the osteoclast marker and toluidine blue staining for imaging bone-attached osteoblasts (Romeo et al., 2019; Colaianni et al., 2015). Osteoclast and osteoblast density did not significantly differ between the SMN2 1-copy mutant and the control (Figure 1—figure supplement 2C–E). The higher BMD may be attributed to greater mechanical stress caused by the shorter femur supporting the weight of the body, consistent with prior research indicating that elevated mechanical force leads to higher BMD in the femur (Hoxha et al., 2014; Ike et al., 2015). Nevertheless, the decrease in bone growth without apparent deterioration in bone mineralization of the femur of SMN2 1-copy Smn1ΔMPC mutants is consistent with findings from the whole-body SMA mouse model (Hensel et al., 2020). Collectively, these results suggest that mice carrying low copies of SMN2, with the Smn1 gene specifically deleted in MPCs, exhibit bone growth abnormalities, while osteoblast and osteoclast populations show no obvious defects based on our preliminary analyses.
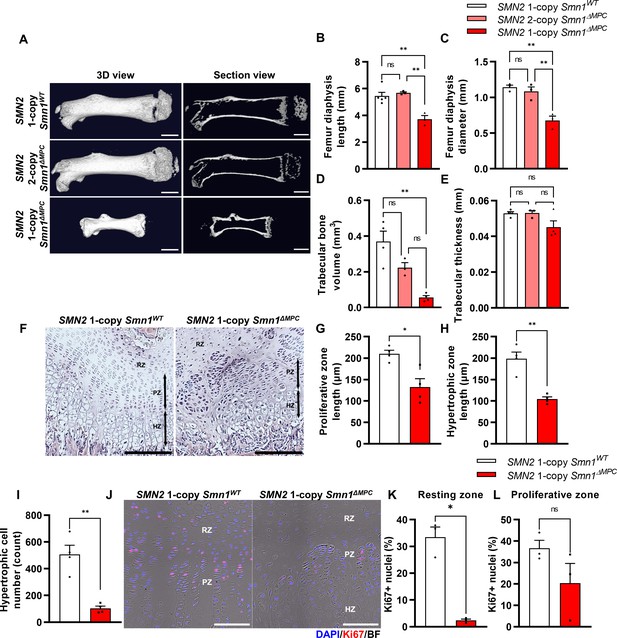
Skeletal growth abnormalities and altered growth plate homeostasis in SMN2 1-copy Smn1ΔMPC mice.
(A) Representative 3D images and longitudinal section view of the ossified femur bone. Scale bars, 1 mm. (B, C) SMN2 1-copy mutant’s femurs showed reduced growth in diaphysis length and diameter, and (D) decreased trabecular bone volume. (E) Trabecular bone thicknesses were not significantly different between the control and mutant groups. The micro-computed tomography (micro-CT) analysis was performed in femur diaphysis and metaphysis from SMN2 1-copy Smn1ΔWT, SMN2 2-copy, and 1-copy Smn1ΔMPC mice at P14. One-way analysis of variance (ANOVA) with Tukey’s post hoc test, n = 3–5 mice in each genotype (B–E). (F) Representative images of hematoxylin and eosin (H&E) staining in the distal femur growth plate of control and mutant mice with 1 copy of SMN2 at P14. Scale bars, 100 μm. Resting zone (RZ), hypertrophic zone (HZ), and proliferative zone (PZ). (G–I) Indicated by black arrows, the HZ and PZ lengths were reduced in SMN2 1-copy Smn1ΔMPC mice, and the hypertrophic cell number in a section of the 1-copy mutant was decreased (n = 4 mice in each genotype; unpaired t-test with Welch’s correction). (J) Representative images of Ki67 immunostaining in the distal femur growth plate of control and mutant mice with 1 copy of SMN2 at P14. Scale bars, 100 μm, and (K) decreased Ki67+ percentage in resting zone chondrocytes. (L) Ki67+ percentage in the proliferative zone was not significantly different between the control and mutant groups. n = 3 mice in each genotype; unpaired t-test with Welch’s correction (K–L). ns: not significantly different. *p < 0.05; **p < 0.01. Error bars show standard error of the mean (SEM).
Various bone-forming cells originating from the LPM were known to contribute to bone formation, such as growth plate chondrocytes and osteoblasts. In SMN2 1-copy Smn1ΔMPC mutants, SMN may be deleted in these cells, suggesting that they play a role in the bone growth abnormalities observed in 2-week-old mice. Previous researchers revealed that primary osteoblasts from a severe SMA mouse model did not display notable differences from controls in an in vitro ossification test. And they did not observe any differences in bone voxel density and bone thickness in femurs at P3 severe SMA mice. This is supported by the absence of any bone thickness or BMD defects in the SMN2 1-copy mutant (Figure 1E and Figure 1—figure supplement 2A, B), and the unimpaired osteoblast population (Figure 1—figure supplement 2E). Thus, we conclude that the bone growth abnormalities observed in the 2-week-old SMN2 1-copy Smn1ΔMPC mutant are due to impaired endochondral ossification.
To determine whether bone growth defects in SMN2 1-copy Smn1ΔMPC mutants arise from disrupted chondrocyte homeostasis at growth plates, we stained the femur distal growth plate of P14 mice with hematoxylin and eosin (H&E; Figure 1F). In line with earlier findings in whole-body SMA mice (Hensel et al., 2020), SMN2 1-copy Smn1ΔMPC mice exhibited shorter proliferative and hypertrophic zones compared to control mice (Figure 1G, H). Additionally, there was a significant reduction in the number of chondrocytes in the hypertrophic zone (Figure 1I). To investigate the decrease in chondrocyte proliferation and subsequent reduction in the proliferative and hypertrophic zone, we stained the proliferation marker Ki67 in the growth plate of both SMN2 1-copy control and mutant samples (Figure 1J). We then quantified the percentage of Ki67+ nuclei in the resting and proliferative zones (Figure 1K, L). Although there was no significant difference observed in the Ki67+ percentage of proliferative zone chondrocytes in the proceeding proliferation state, there was an absolute reduction in resting zone chondrocyte proliferation. The decreased proliferation rate in the resting zone could have impeded the transition to the proliferative zone. Our data indicate that adequate expression of SMN is essential for the homeostasis of chondrocytes at growth plates.
Disruption of chondrocyte-derived IGF signaling in SMN2 1-copy Smn1ΔMPC mutants
The proliferation and differentiation of growth plate chondrocytes are regulated by systemic IGF (Shim, 2015; Karimian et al., 2011; Racine and Serrat, 2020). Previous research suggested that a key factor contributing to the pathological phenotype in SMA is the lowered expression of the Igf1/Igfbp3/Igfals genes, which produce IGF and IGF-carrying proteins, in the liver (Hua et al., 2011; Murdocca et al., 2012). As the IGF pathway proteins are downregulated in whole-body SMA mice, the bone growth defects observed in the mice have sparked debate as it remains unclear whether they are due to cell-autonomous defects by bone-forming cells’ SMN reduction, or the low liver-derived IGF level (Hua et al., 2011; Tsai et al., 2014; Deguise et al., 2021; Hensel et al., 2020).
To clarify this issue, we used Smn1ΔMPC mutant mice, which enabled us to investigate the effect of SMN depletion in bone-forming cells such as chondrocytes on bone growth, while circumventing the impact of the endocrine signal by Prrx1-negative organ. To investigate the impact of IGF signaling on growth plate chondrocytes, we employed immunofluorescence to evaluate the percentage of p-AKT-positive cells activated by the IGF–PI3K–AKT pathway in both SMN2 1-copy control and mutant femur distal growth plate (Figure 2A). Intriguingly, the percentage of p-AKT+ cells was significantly decreased in resting zone chondrocytes, but not in the proliferative zone, which aligns with the Ki67+ percentage (Figure 2B, C). Expectedly, the liver’s mRNA expression of IGF pathway genes, reported to be decreased in SMA mouse models and patients (Hua et al., 2011; Murdocca et al., 2012; Deguise et al., 2021; Sahashi et al., 2013), showed no difference when comparing controls to SMN2 1-copy Smn1ΔMPC mutants (Figure 2D). These findings indicate that the growth plate’s proliferation and hypertrophy in SMN2 1-copy mutants are affected by impairments in another AKT upstream signal rather than by liver-secreted systemic IGF.

Decreased chondrocyte-derived IGF–AKT axis by limb mesenchymal cell-specific survival motor neuron (SMN) depletion in SMN2 1-copy Smn1ΔMPC mice.
(A) Representative images of p-AKT immunostaining in distal femur growth plate from mice at P14. Scale bars, 100 μm. (B, C) The p-AKT-positive percentage was decreased in the resting zone chondrocytes, not in the proliferative zone (n = 3–4 mice in each genotype; unpaired t-test with Welch’s correction). (D) Relative IGF axis mRNA expression in the livers of SMN2 1-copy control and mutant mice. The IGF pathway genes showed no difference when comparing controls to SMN2 1-copy Smn1ΔMPC mutants. (E, F) Relative IGF axis and chondrocyte differentiation marker mRNA expression in the chondrocytes of SMN2 1-copy control and mutant mice. The Igf1, Igfbp3, and hypertrophic marker Col10a1 expression were decreased in SMN2 1-copy Smn1ΔMPC mutants. n = 3 mice in each genotype; unpaired t-test with Welch’s correction (D–F). (G) Representative images of genomic PCR analysis from SMN2 1-copy Smn1ΔMPC mice tissues at P21. (H) Quantitative reverse transcription polymerase chain reaction (qRT-PCR) analysis from tissues of SMN2 1-copy Smn1WT and Smn1ΔMPC mice at P21 (n = 3 mice in each genotype; unpaired t-test with Welch’s correction). Deletion of Smn1 exon 7 was detected only in limb mesenchymal cells using genomic PCR (G) and full-length Smn1 mRNA expression (H). (I) Representative images of western blot analysis in cultured fibro-adipogenic progenitors (FAPs). SMN protein in FAPs of SMN2 1-copy Smn1ΔMPC mice exhibited a decrease comparable to that observed in the SMAΔ7 mice. (J) Relative SMN levels in cultured FAPs of the controls and mutants (n = 3 mice in each genotype; one-way analysis of variance (ANOVA) with Tukey’s post hoc test). ns: not significantly different. *p < 0.05; **p < 0.01; ***p < 0.001; ****p < 0.001. Error bars show standard error of the mean (SEM).
-
Figure 2—source data 1
Original file for the gel electrophoresis of genomic PCR in Figure 2G (Cre, Smn1F7, Smn1Δ7) and western blot analysis in Figure 2I (anti-alpha-tubulin, anti-SMN).
- https://cdn.elifesciences.org/articles/92731/elife-92731-fig2-data1-v1.zip
-
Figure 2—source data 2
PDF containing Figure 2G, I and original scans of the PCR and western blot with highlighted bands and sample labels.
- https://cdn.elifesciences.org/articles/92731/elife-92731-fig2-data2-v1.zip
There are reports indicating that local IGF expression plays a crucial role in bone development through the growth plate, in addition to circulating IGF (Hallett et al., 2019; Racine and Serrat, 2020). While most Igf1-null mice died before birth and had smaller tibial lengths than normal, liver-specific Igf1-deleted mice did not experience significant changes in body length or tibial length during postnatal growth, despite a 75% reduction in serum IGF-1 levels (Baker et al., 1993; Yakar et al., 1999). This indicates that local IGF in the growth plate is crucial for endochondral ossification, in addition to serum IGF. The chondrocyte-specific Igf1 knockout mouse demonstrated a reduction in postnatal body and femur length, and the chondrocyte-specific Igf1r knockout mouse demonstrated a significant reduction in bone growth, as well as a decrease in both growth plate proliferative and hypertrophic zone (Govoni et al., 2007; Wang et al., 2011). A recent study revealed that resting zone chondrocytes in the growth plate serve as a major source of local IGF and activate the p-AKT pathway via autocrine and paracrine IGF signaling (Oichi et al., 2023). The study further revealed that cells that constitute bone and bone marrow, apart from chondrocytes, do not express Igf1, making chondrocytes the solitary source of local IGF. These suggest that the growth plate defects and the reduction of resting zone AKT phosphorylation in SMN2 1-copy mutants may be due to chondrocyte-secreted IGF deficiency. To confirm this hypothesis, we evaluated the expression of IGF-related genes in chondrocytes from both SMN2 1-copy control and mutant femur (Figure 2E). Indeed, Igf1 and Igfbp3 were greatly depleted in SMN2 1-copy mutant chondrocytes. It is hypothesized that the increased presence of Ghr may be in response to the reduction of Igf1. As IGF directly causes chondrocyte hypertrophy (Wang et al., 1999), we assess the mRNA expression of chondrocyte hypertrophic marker Col10a1 and undifferentiated chondrocyte marker Col2a1 (Figure 2F). The results show that Col10a1 is decreased, while Col2a1 is increased in the mutant. In Figure 1H, I, the hypertrophic cell reduction may be caused by a low local IGF level. Therefore, depletion of local IGF in the growth plate of SMN2 1-copy mutants may hinder chondrocyte progression to proliferation and hypertrophy, leading to aberrations in endochondral ossification. In Figures 1L and 2C, it is possible that the serum IGF could affect the remnant proliferative and p-AKT-positive cells in the growth plate via the vascularization of bone marrow. Based on these findings, it was concluded that deprivation of SMN in chondrocytes leads to a decrease in local IGF signaling, which affects growth plate homeostasis.
Mesenchymal cell-specific SMN reduction similar to severe SMA mouse model in SMN2 1-copy Smn1ΔMPC mutants
To confirm the specific deletion of Smn1 in limb mesenchymal cells, including chondrocytes and FAPs, we performed quantitative reverse transcription polymerase chain reaction (qRT-PCR) for full-length Smn1 mRNA expressed by undeleted Smn1 allele. Our findings indicate that full-length Smn1 mRNA expression in the brain, liver, skeletal muscle, and spinal cord of SMN2 1-copy Smn1ΔMPC mice at postnatal day 21 (P21) was similar to that of control mice, while it was significantly reduced in isolated FAPs and chondrocytes (Figure 2H). Additionally, we confirmed the presence of the Smn1Δ7 variant, which is the exon 7-deleted form of Smn1 created by cre-lox-mediated recombination, in both FAPs and chondrocytes by conducting genomic PCR on the SMN2 1-copy Smn1ΔMPC mutant (Figure 2G). Since SMN was not downregulated in the tissues other than limb mesenchymal cells, non-mesenchymal cells were ruled out from being responsible for the phenotype observed in Smn1ΔMPC mutants.
Among our Smn1ΔMPC models, mice with two copies of SMN2 exhibit similar bone development parameters as control mice without SMN deletion. However, mice with one copy of SMN2 display bone pathological defects akin to SMA mouse models. This may be because the quantity of human full-length SMN protein produced by SMN2 2-copy, was sufficient to sustain SMN complex function in the SMN2 2-copy Smn1ΔMPC mutants, despite the absence of functional mouse SMN protein in limb mesenchymal cells. On the contrary, due to the lower expression of full-length SMN compared to SMN2 2-copy mutants, the SMN complex may not function properly in SMN2 1-copy mutant cells. We confirmed this by comparing the amount of full-length SMN protein in isolated FAPs from the hindlimbs of control, Smn1ΔMPC mutants, and a severe SMA mouse model (Smn1−/−; SMN2+/+; SMNΔ7+/+; SMAΔ7 mutants) (Figure 2I). The data show that SMN2 1-copy Smn1ΔMPC mutants exhibited ~80% reduction in SMN levels compared to the control group. The level of SMN protein in SMN2 1-copy Smn1ΔMPC mutants was similar to that in SMAΔ7 mutants. Conversely, SMN2 2-copy mutants display a decrease of approximately 40% in SMN protein levels compared to the control (Figure 2J). The moderately reduced expression of SMN is adequate to support regular bone development in SMN2 2-copy mutants. Taken together, our findings indicate that the reduction of mesenchymal SMN to levels comparable to that of the severe SMA mouse model causes SMA-like bone pathology in the SMN2 1-copy mutant.
Abnormal NMJ maturation in SMN2 1-copy Smn1ΔMPC mutants
To investigate whether disabling SMN in FAPs results in SMA-like neuromuscular impairments, we assessed if NMJ phenotypes observed in SMA mouse models also occur in SMN2 1-copy Smn1ΔMPC mutants. Both severe and mild SMA mouse models exhibit impaired NMJ maturation markers, including plaque-like morphology of acetylcholine receptor (AChR) clusters, neurofilament (NF) varicosities, and poor terminal arborization (Kong et al., 2009; Martinez et al., 2012; Monani et al., 2003; Kariya et al., 2008). To evaluate the impact of SMN deficiency in FAPs on NMJ maturation, we evaluated the NMJ maturation markers in the tibialis anterior (TA) muscles of control and SMN2 1-copy Smn1ΔMPC mutant mice at P21, a time when NMJ maturation is in progress (Figure 3A). Our examination revealed the presence of NF varicosities in SMN2 1-copy mutants as compared with control mice (Figure 3A, D). Additionally, the number of nerve branches was decreased and half of the total NMJs were poorly arborized in SMN2 1-copy mutants (Figure 3B, C). These presynaptic alterations are specific phenotypes in neurogenic atrophy-like SMA. Unlike neurogenic atrophy, physiologic atrophy shows no differences in presynaptic morphology, such as nerve branching (Deschenes et al., 2006). This suggests that the NMJ phenotypes observed in SMN2 1-copy Smn1ΔMPC mutant mice are not caused by decreased muscle size and activity resulting from bone growth abnormalities. The morphology of AChR clusters shows that the mutants have more immature plaque-like NMJs than the controls’ pretzel-like structure (Figure 3E). Therefore, these findings indicate that SMN2 1-copy Smn1ΔMPC mutants exhibit NMJ maturation abnormalities common in SMA mouse models.
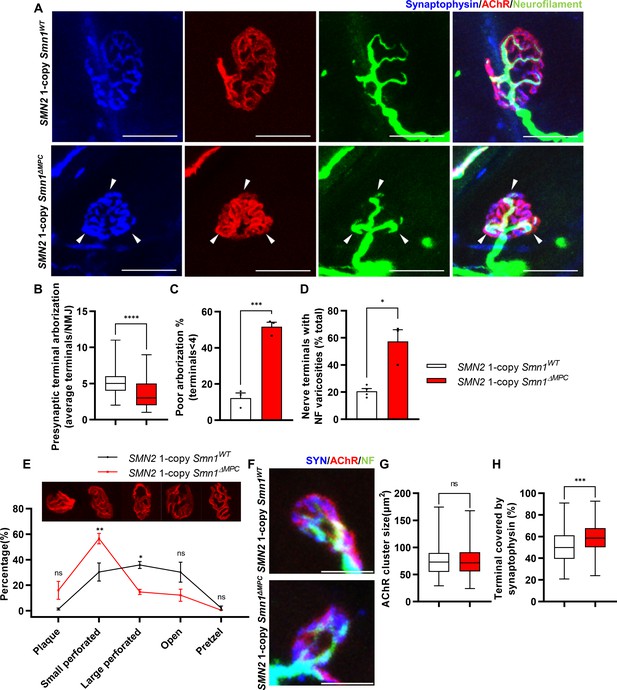
Aberrant postnatal neuromuscular junction (NMJ) maturation in SMN2 1-copy Smn1ΔMPC mice.
(A) Immunostaining of NMJs in TA muscle of SMN2 1-copy Smn1WT and Smn1ΔMPC mice at P21 with anti-NF (green), anti-synaptophysin (blue), and α-Btx staining acetylcholine receptor (AChR; red). Scale bars, 20 μm. The confocal images of NMJs showed decreased presynaptic terminal branching and the existence of nerve terminal varicosities that were enlarged with neurofilament (NF; indicated by arrowheads) in the mutant. (B) The NMJs of the SMN2 1-copy mutant exhibited a significant decrease in presynaptic terminal arborization and (C) an increased percentage of poorly arborized NMJs (n = 3 mice in each genotype; unpaired t-test with Welch’s correction). (D) The percentage of NMJs exhibiting NF varicosities was higher in the SMN2 1-copy mutant group than in the control group (n = 3–4 mice in each genotype; unpaired t-test with Welch’s correction). (E) For quantification of the NMJ maturation stage, we classified NMJs into five distinct developmental stages (Plaque: plaque-shaped endplate without any perforation; Small perforated: plaque-shaped endplate with small perforations; Large perforated: plaque-shaped endplate with large perforations; Open: C-shaped endplate; Pretzel: pretzel-like shaped endplate) and then compared the frequency patterns of SMN2 1-copy control and mutant mice (n = 3 mice in each genotype; two-way analysis of variance (ANOVA) with Tukey’s post hoc test). The NMJs of SMN2 1-copy mutants displayed plaque-like shapes, indicating that they were in the immature stage. (F) Immunostaining of NMJs in TA muscle of SMN2 1-copy Smn1WT and Smn1ΔMPC mice at P3 with anti-NF (green), anti-synaptophysin (blue), and α-Btx staining AChR (red). Scale bars, 10 μm. (G) There were no significant differences in AChR cluster size between the SMN2 1-copy control and mutant at P3 (n = 3–4 mice in each genotype; unpaired t-test with Welch’s correction). (H) The ratio of the Synaptophysin area to the AChR area in NMJ was slightly higher in the SMN2 1-copy mutant at P3 (n = 3–4 mice in each genotype; unpaired t-test with Welch’s correction). ns: not significantly different. *p < 0.05; **p < 0.01; ***p < 0.001; ****p < 0.0001. All box-and-whisker plots show the median, interquartile range, minimum, and maximum. For the box-and-whisker plots, range bars show minimum and maximum (B, G, H). For the bar and line graph, error bars show standard error of the mean (SEM) (C–E).
Undisturbed NMJ formation in neonatal SMN2 1-copy Smn1ΔMPC mutants
To determine whether any NMJ defects were present prior to juvenile NMJ maturation in SMN2 1-copy Smn1ΔMPC, we examined NMJ formation in SMN2 1-copy Smn1ΔMPC mice at the neonatal stage on postnatal day 3. We evaluated the AChR and nerve terminal areas to assess post- and presynaptic development, respectively (Figure 3F). Measurements of AChR cluster size indicated no differences between control and SMN2 1-copy Smn1ΔMPC mice (Figure 3G). However, the area of AChR covered by nerve terminals was slightly larger in SMN2 1-copy Smn1ΔMPC (Figure 3H). We have no reasonable explanation for why the coverage is higher in the mutant. However, there does not appear to be abnormal development of the NMJ in the mutant, at least until the neonatal period. Therefore, we reasoned that SMN2 1-copy Smn1ΔMPC mutants began to exhibit deterioration in the NMJ maturation during the juvenile stage, following the intact neonatal development of NMJ.
Aberrant NMJ morphology in the adult SMN2 1-copy Smn1ΔMPC mice
To evaluate the organization of NMJ after the conclusion of postnatal NMJ development, considering mesenchymal SMN expression, we examined NMJ morphology in the TA muscle of control, SMN2 1-copy, and 2-copy Smn1ΔMPC mice at postnatal day 56 (P56). Our analysis revealed that presynapses were fragmented in SMN2 1-copy mutants, resulting in a bouton-like morphology, in contrast to the control and SMN2 2-copy Smn1ΔMPC mice (Figure 4A). In SMN2 1-copy Smn1ΔMPC mice, a twofold presynaptic fragmentation compared to the control was quantified, demonstrating nerve terminal shrinkage (Figure 4B). Additionally, NF ends displayed more severe varicosities than at P21 and were only connected to the proximal nerve by very thin NF, unlike control and SMN2 2-copy mice (Figure 4C). Remarkably, numerous presynaptic islands formed in SMN2 1-copy Smn1ΔMPC mice through the merging of fragmented presynapses and NF varicosity. In SMN2 1-copy mutants, AChR clusters displayed fragmented grape-shaped morphology that overlapped with nerve terminals, whereas control and SMN2 2-copy mice displayed pretzel-like structures (Figure 4D). These results suggest that defects in adult NMJ morphology occur when mesenchymal SMN protein is reduced to the extent of the SMN2 1-copy Smn1ΔMPC mutants.

Morphological deterioration in neuromuscular junctions (NMJs) of adult SMN2 1-copy Smn1ΔMPC mice.
(A) Immunostaining of NMJs in TA muscle of SMN2 1-copy Smn1WT, SMN2 2-copy, and SMN2 1-copy Smn1ΔMPC mice at P56 with anti-NF (green), anti-synaptophysin (blue), and α-Btx staining acetylcholine receptor (AChR; red). Scale bars, 20 μm. The confocal images of NMJs showed fragmentation and bouton-like neurofilament (NF) varicosities (indicated by arrowheads) in the SMN2 1-copy Smn1ΔMPC mice. The NMJs of the SMN2 1-copy mutant displayed fragmented presynapse (B), endplate (D), and NF varicosities (C) compared to SMN2 1-copy Smn1WT and SMN2 2-copy Smn1ΔMPC mice (n = 3–5 mice in each genotype; Presynaptic fragments and AChR fragments: Brown–Forsythe and Welch analysis of variance (ANOVA) with Games–Howell’s test; NF varicosities: one-way ANOVA with Tukey’s post hoc test). ns; not significantly different. ****p < 0.0001. All box-and-whisker plots show the median, interquartile range, minimum, and maximum. For the box-and-whisker plots, range bars show minimum and maximum (B, D). For the bar graph, error bars show standard error of the mean (SEM) (C).
Presynaptic neurotransmission alteration in SMN2 1-copy Smn1ΔMPC mutants
To investigate whether the morphologically aberrant NMJs of SMN2 1-copy Smn1ΔMPC mice have functional impairments, we isolated hindlimb extensor digitorum longus (EDL) muscles from P56 mice and conducted electrophysiological recording. We incubated the muscles with μ-conotoxin, which selectively inhibits muscle voltage-gated Na+ channels, prevented the induction of muscle action potential (Ling et al., 2010; Zanetti et al., 2018), and recorded the Miniature endplate potential (mEPP) and evoked endplate potential (eEPP) (Ling et al., 2010; Zanetti et al., 2018). mEPP, a response that occurs when spontaneously released acetylcholine binds to nicotinic AChR without nerve stimulation, was measured ex vivo near the NMJs of the EDL muscles in the control and SMN2 1-copy Smn1ΔMPC mice (Figure 5A). The mEPP amplitude was increased in SMN2 1-copy Smn1ΔMPC mice (Figure 5B), whereas mEPP frequency was comparable between the controls and mutants (Figure 5C). The results indicate that the NMJ synapses of SMN2 1-copy Smn1ΔMPC mice are functional and more sensitive to acetylcholine compared to the controls. Next, we measured the eEPP by stimulating an action potential at the peroneal nerve (Figure 5D). Despite the increased mEPP amplitude, the amplitude of eEPPs was significantly decreased in SMN2 1-copy Smn1ΔMPC mice (Figure 5E). These results suggest that the nerve terminals in SMN2 1-copy Smn1ΔMPC mice exhibit decreased quantal content. This could be due to a decrease in vesicle release probability. Notably, there was no difference in the paired-pulse response, indicating normal neurotransmitter release probability (Figure 5F, G). Taken together, these findings suggest that the presynaptic neurotransmission ability of the NMJ is reduced in SMN2 1-copy Smn1ΔMPC mutants.

Reduced presynaptic neurotransmission ability in the neuromuscular junctions (NMJs) of SMN2 1-copy Smn1ΔMPC mice.
(A) Representative traces of Miniature endplate potential (mEPP) from SMN2 1-copy Smn1ΔWT (top) and SMN2 1-copy Smn1ΔMPC (bottom) mice. (B, C) SMN2 1-copy mutant’s NMJs showed an increase in mEPP amplitude and no differences in mEPP frequency (1-copy control, n = 25, 9 mice; 1-copy mutant, n = 21, 8 mice; unpaired t-test with Welch’s correction). (D) Representative traces of evoked endplate potential (eEPP) from SMN2 1-copy Smn1ΔWT (top) and SMN2 1-copy Smn1ΔMPC (bottom) mice. (E) The mutant’s NMJs showed a stronger amplitude of eEPPs (1-copy control, n = 12, 4 mice; 1-copy mutant, n = 12, 3 mice; unpaired t-test with Welch’s correction). (F) Representative traces of paired-pulse response from SMN2 1-copy Smn1ΔWT (top) and SMN2 1-copy Smn1ΔMPC (bottom) mice. (G) Paired-pulse response was not different between SMN2 1-copy control and mutant NMJs, indicating a comparable neurotransmitter release probability (1-copy control, n = 8, 3 mice; 1-copy mutant, n = 6, 3 mice; unpaired t-test with Welch’s correction). The electrophysiological recording was performed in the extensor digitorum longus (EDL) muscle at P56. ns: not significantly different. *p < 0.05. All box-and-whisker plots show the median, interquartile range, minimum, and maximum. For the box-and-whisker plots, range bars show minimum and maximum (B, C, E, G).
Disturbed nerve terminal structure in SMN2 1-copy Smn1ΔMPC mice
To examine the NMJ ultrastructure of SMN2 1-copy Smn1ΔMPC mutants, we utilized transmission electron microscopy (TEM) (Figure 6A). The density of junctional folds in SMN2 1-copy Smn1ΔMPC mutant specimens was comparable to that of the control (Figure 6B). However, the density of synaptic vesicles was substantially elevated in the SMN2 1-copy mutants (Figure 6C). Since previous electrophysiological results suggested a decrease in presynaptic neurotransmission capacity in SMN2 1-copy mutants, this could be due to synaptic vesicles failing to fuse with the membrane, leading to the accumulation of vesicles in the terminal and reduced quantal contents in Figure 5. In Figure 3H, larger synaptophysin coverage in the mutant may be caused by this synaptic vesicle accumulation. Additionally, the detachment of the nerve terminal is more frequent at the NMJ of mutants (Figure 6D). The detachment of nerve terminals observed in SMN2 1-copy Smn1ΔMPC mutants could have also resulted in diminished presynaptic neurotransmission capacity. Collectively, these findings indicate that SMN2 1-copy Smn1ΔMPC mutants have nerve terminal-specific pathological defects at the NMJ ultrastructural level.

Abnormal nerve terminal ultrastructure in SMN2 1-copy mutant.
(A) Representative transmission electron microscopy (TEM) images from neuromuscular junctions (NMJs) of SMN2 1-copy Smn1ΔWT and SMN2 1-copy Smn1ΔMPC mice at P56. Scale bars, 500 nm. Nerve terminal (NT; indicated by the blue zone). Synaptic vesicles (SVs). Muscle fiber (MS; indicated by the red zone). Endplate junctional folds (JF). Nerve terminal detachment (indicated by arrow) was observed in SMN2 1-copy Smn1ΔMPC mice. (B) The density of junctional folds in the NMJ of SMN2 1-copy Smn1ΔMPC mice no significant change compared to the control, whereas (C) the density of synaptic vesicles was increased (n = 3–4 mice in each genotype; unpaired t-test with Welch’s correction). (D) The detachment of the nerve terminal occurs more frequently at the NMJ of mutants (n = 3–4 mice in each genotype; unpaired t-test with Welch’s correction). ns: not significantly different. *p < 0.05; ***p < 0.001. All box-and-whisker plots show the median, interquartile range, minimum, and maximum. For the box-and-whisker plots, range bars show minimum and maximum (B, C). For the bar graph, error bars show standard error of the mean (SEM) (D).
FAPs transplantation rescues NMJ morphology in limb mesenchymal SMN mutants
SMN-deleted limb mesenchymal tissues in SMN2 1-copy Smn1ΔMPC mutants comprise not only FAPs, but also bone, cartilage, pericytes, and tendon, among others (Leinroth et al., 2022; Nassari et al., 2017). To evaluate the critical role of FAPs in the postnatal development of the NMJ, we isolated fluorescent protein-labeled wild-type FAPs from Prrx1Cre; Rosa26LSL-YFP/+ or Prrx1Cre; Rosa26 LSL-tdTomato/+ mice and transplanted them into the TA muscles of SMN2 1-copy Smn1ΔMPC mice at postnatal day 10. The TA muscle on the contralateral side was treated with a vehicle as a control. In SMN2 1-copy Smn1ΔMPC mice at P56, the muscle that received FAPs showed decreased presynaptic fragmentation, NF varicosities, and postsynaptic fragmentation compared to the contralateral muscle (Figure 7A–D). As a result, the transplanted muscle was not significantly different from the control except for NF varicosities. These data demonstrate that the transplantation of wild-type FAPs rescues the abnormal NMJ development in SMN2 1-copy Smn1ΔMPC mice. Overall, our findings indicate that SMN depletion in FAPs leads to the neuronal SMN-independent NMJ pathology in severe SMA, which is rescued by the transplantation of healthy FAPs.
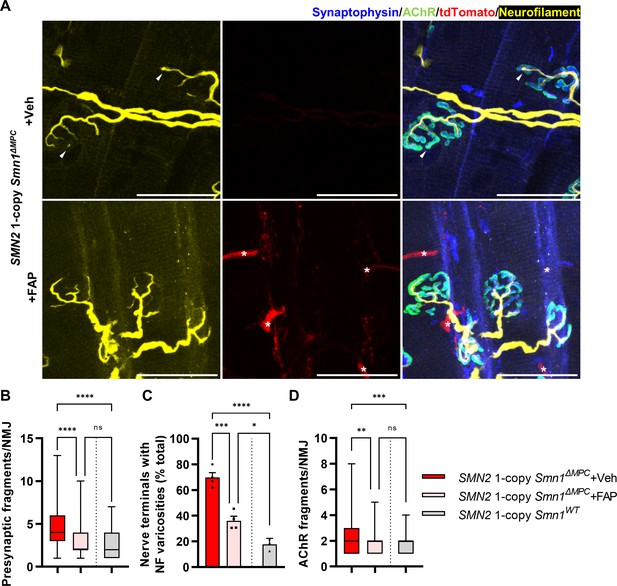
Improved postnatal neuromuscular junction (NMJ) development in the TA muscle of SMN2 1-copy Smn1ΔMPC mice following healthy fibro-adipogenic progenitors (FAPs) transplantation.
(A) Immunostaining of NMJs in tdTomato+ FAP-transplanted TA muscle (+FAP) and vehicle-treated contralateral muscle (+Veh) in SMN2 1-copy Smn1ΔMPC mice at P56 with anti-NF (yellow), anti-synaptophysin (blue), α-Btx staining acetylcholine receptor (AChR; green), and tdTomato fluorescence (red). Scale bars, 40 μm. The images revealed neurofilament (NF) varicosities (indicated by arrowheads) in the +Veh NMJs. The tdTomato+ FAPs (marked by asterisks) were transplanted into +FAP NMJs, which exhibited (B) decreased presynaptic fragmentation, (C) NF varicosities, and (D) AChR fragmentation compared to +Veh NMJs and similar to wild-type NMJs (n = 3–4 mice in each group; unpaired t-test with Welch’s correction). ns: not significantly different. *p < 0.05, **p < 0.01, ***p < 0.001, ****p < 0.0001. All box-and-whisker plots show the median, interquartile range, minimum, and maximum. For the box-and-whisker plots, range bars show minimum and maximum (B, D). For the bar graph, error bars show standard error of the mean (SEM) (C).
Discussion
In this paper, we elucidate the contribution of SMN depletion in mesenchymal progenitors for the pathogenesis of SMA. To test this hypothesis, we generated conditional knockout mouse strains to delete the Smn1 allele specifically in limb mesenchymal cells and carry human SMN2 copies. Our research using these mouse models resulted in three major discoveries. First, SMN deficiency in FAPs contributes to NMJ pathological defects in SMA. We observed delayed NMJ maturation and varicosities in juvenile SMN2 1-copy Smn1ΔMPC mutant. The pathogenic NMJ phenotypes were also observed in the SMAΔ7 mutant, which is one of the severe SMA mouse models (Kong et al., 2009; Martinez et al., 2012; Kariya et al., 2008). As the SMAΔ7 mutant typically lives for approximately 12 days, the fragmentation of the NMJ in adult SMN2 1-copy mutant was not evaluated in the severe SMA mutant. Nevertheless, models that induce a conditional adult SMN deficiency through either CreER allele or oligonucleotide administration resulting in SMN reduction, demonstrated the depletion of SMN throughout the body caused fragmentation of NMJ (Sahashi et al., 2013; Kariya et al., 2014). Thus, we demonstrate that the SMN2 1-copy Smn1ΔMPC mutant model mimics whole-body SMA mouse models in NMJ morphology. In the electrophysiological test, the SMAΔ7 mutant exhibited reduced quantal content, readily releasable pool, and vesicle release probability (Torres-Benito et al., 2011). In our study, we did not directly assess the readily releasable pool in SMN2 1-copy Smn1ΔMPC mutant by stimulus train of electrophysiological recording but instead showed reduced quantal content and normal vesicle release probability. In previous studies, it was theorized that the decrease in quantal contents in SMAΔ7 mutants resulted from decreased synaptic vesicle density at nerve terminals caused by motor neuron defects and abnormal axonal transport (Dale et al., 2011; Kong et al., 2009). However, we found that synaptic vesicle density was increased in the SMN2 1-copy Smn1ΔMPC mutant with SMN-sufficient motor neurons. It is possible that the alteration of active zones, which were also altered in the SMA motor terminals (Kong et al., 2009), contributed to the reduction in synaptic vesicle fusion and the decreased quantal contents. Indeed, nerve terminal detachment in SMN2 1-copy mutant mice was also found in active zone complex protein integrin-α3 knockout mice (Ross et al., 2017). Based on the rescue data of transplanted healthy FAPs, we can report that FAP-specific SMN depletion is involved in NMJ pathology of SMA.
Second, we demonstrated that skeletal growth defects, a phenotype observed in SMA (Khatri et al., 2008; Vai et al., 2015; Wasserman et al., 2017; Baranello et al., 2019; Hensel et al., 2020), are a cell-autonomous pathological effect of the depletion of SMN in bone-forming cells. We observed reduced bone size and volume in juvenile SMN2 1-copy Smn1ΔMPC mutant. Depletion of SMN in chondrocytes showed growth plate homeostasis problems with chondrocyte-secreted IGF defects. However, our skeletal study has a limitation in that we did not assess potential impairment of endochondral ossification during embryonic development. It may be necessary to further evaluate the embryonic period in addition to the postnatal growth plate defects observed in our study, as cells of the Prrx1-lineage are involved in mesenchymal condensation and chondrocyte differentiation during embryonic endochondral ossification (Hallett et al., 2019; Racine and Serrat, 2020). Previous studies have reported that IGF1 overexpression improves biochemical and behavioral manifestations in SMA mice, suggesting potential therapies for SMA (Bosch-Marcé et al., 2011; Tsai et al., 2014). Our study showed that these IGF therapies for SMA could be one to consider for treating bone growth abnormalities. In addition, the skeletal growth defect may affect physiologic muscle atrophy through imbalanced muscle contraction and reduced tension. This physiologic atrophy may be part of SMA-associated muscle weakness independent of neurogenic atrophy.
Third, it was demonstrated that adequate levels of SMN protein are essential for MPCs to contribute to limb neuromusculoskeletal development. The mutants with only 1 copy of SMN2 exhibit problematic symptoms observed in both SMA patients and mouse models, while SMN2 2-copy mutants display a typical phenotype in bone and NMJ. The lack of SMN protein in MPCs by insufficient SMN2 copies, similar to the deficiency seen in severe SMA, is responsible for the onset of SMA pathology. Thus, we propose that restoration of deficient SMN in MPCs is crucial for rehabilitating their function. Based on these discoveries, SMN replenishment treatments for MPCs, specifically FAPs, and chondrocytes, are necessary to provide a complete solution for neuromusculoskeletal defects in severe SMA patients.
The initial focus for the treatment of SMA was on motor neurons located in the spinal cord, and pharmaceuticals were created to address the deficiency of SMN in these neurons (Mercuri et al., 2020). For example, intrathecal injections of Spinraza can efficiently boost SMN in the CNS, including the spinal cord (Passini et al., 2011; Claborn et al., 2019). However, Spinraza does not address the lack of SMN in peripheral tissues, including mesenchymal cells, highlighted in recent studies. The drug Zolgensma, which employs AAV9 to express SMN through systemic delivery, appears to resolve this issue (Foust et al., 2009; Valori et al., 2010; Mattar et al., 2013). Nevertheless, a prior study indicates that chondrocytes within the growth plate and articular cartilage do not get infected with AAV9 (Yang et al., 2019). Furthermore, it is well established that DNA vectors delivered via AAV9 undergo dilution during cell proliferation (Penaud-Budloo et al., 2008; Colella et al., 2018; Van Alstyne et al., 2021; Heller et al., 2021). Given the infection issue and the dilution issue by the active cell population changes of chondrocytes and FAPs during postnatal development (Petrany et al., 2020; Bachman and Chakkalakal, 2022), SMN supplementation through Zolgensma alone would not be sufficient for severe SMA patients. Thus, there is an urgent need to research and develop therapeutic strategies that target mesenchymal progenitors.
While this study is the first to demonstrate the impact of SMN depletion in FAPs on the NMJ development, it does not elucidate the specific mechanism by which FAPs influence the NMJ. Our study observed NMJ recovery in the muscles transplanted with healthy FAPs, but not in the contralateral muscles, indicating that FAPs are likely involved in NMJ development through juxtacrine or paracrine signaling. Since FAPs interact with surrounding tissues through a variety of signaling factors, such as extracellular matrix (Contreras et al., 2021; Scott et al., 2019), Wnt-related protein (Lukjanenko et al., 2019), and Bmp signaling protein (Uezumi et al., 2021; Camps et al., 2020), mis-splicing of the signaling factors due to SMN reduction could disturb the homeostasis of neighboring tissues (Zhang et al., 2008). Furthermore, the Hsd11b1-positive subpopulation of FAPs associated with NMJ was discovered through single-cell RNA sequencing in a recent study (Leinroth et al., 2022). This population is located adjacent to the NMJ and responds to denervation, indicating an increased possibility of interaction with the NMJ organization. Therefore, it is necessary to conduct additional investigations into the expression of various signaling factors by diverse FAP subpopulations in future studies.
Methods
Reagent type (species) or resource | Designation | Source or reference | Identifiers | Additional information |
---|---|---|---|---|
Genetic reagent (Mus musculus) | Prrx1Cre | The Jackson Laboratory | Strain #: 005584; RRID:IMSR_JAX:005584 | |
Genetic reagent (M. musculus) | Smn1f7/+ | The Jackson Laboratory | Strain #: 006138; RRID:IMSR_JAX:006138 | |
Genetic reagent (M. musculus) | Rosa26LSL-YFP/+ | The Jackson Laboratory | Strain #: 006148; RRID:IMSR_JAX:006148 | |
Genetic reagent (M. musculus) | Rosa26LSL-tdTomato/+ | The Jackson Laboratory | Strain #: 007914; RRID:IMSR_JAX:007914 | |
Genetic reagent (M. musculus) | SMN2+/+; Smn1+/− | The Jackson Laboratory | Strain #: 005024; RRID:IMSR_JAX:005024 | |
Genetic reagent (M. musculus) | Smn1+/−; SMN2+/+; SMNΔ7+/+ | The Jackson Laboratory | Strain #: 005025; RRID:IMSR_JAX:005025 | |
Antibody | anti-SMN (Mouse monoclonal) | BD Biosciences | Cat. #: 610646; RRID:AB_397973 | WB (1:1000) |
Antibody | anti-alpha-tubulin (Rabbit monoclonal) | Abcam | Cat. #: ab176560; RRID:AB_2860019 | WB (1:1000) |
Antibody | anti-Neurofilament M (Rabbit polyclonal) | Merckmillipore | Cat. #: ab1987; RRID:AB_91201 | IF (1:1000) |
Antibody | anti-Synaptophysin 1 (Guinea pig polyclonal) | Synaptic Systems | Cat. #: 101 004; RRID:AB_1210382 | IF (1:500) |
Antibody | anti-GFP (Chicken polyclonal) | Abcam | Cat. #: ab13970; RRID:AB_300798 | IF (1:500) |
Antibody | anti-Ki67 (Rabbit polyclonal) | Abcam | Cat. #: ab15580; RRID:AB_443209 | IF (1:500) |
Antibody | anti-Itgb3 (Rabbit monoclonal) | Cell Signaling | Cat. #: 13166; RRID:AB_2798136 | IF (1:100) |
Antibody | anti-p-AKT(S473) (Rabbit monoclonal) | Cell Signaling | Cat. #: 4060; RRID:AB_2315049 | IF (1:100) |
Antibody | anti-CD45-APC (Rat monoclonal) | Biolegend | Cat. #: 103111; RRID:AB_312976 | FACS (3 µl per test) |
Antibody | anti-CD31-APC (Rat monoclonal) | Biolegend | Cat. #: 102409; RRID:AB_312904 | FACS (3 µl per test) |
Antibody | anti-Sca-1(Ly6a)-FITC (Rat monoclonal) | Biolegend | Cat. #: 122507; RRID:AB_756192 | FACS (3 µl per test) |
Antibody | anti-Sca-1(Ly6a)-Pacific blue (Rat monoclonal) | Biolegend | Cat. #: 108120; RRID:AB_493273 | FACS (3 µl per test) |
Antibody | anti-Vcam1-Biotin (Rat monoclonal) | Biolegend | Cat. #: 105703; RRID:AB_313204 | FACS (3 µl per test) |
Antibody | anti-Rabbit IgG-HRP (Goat monoclonal) | Promega | Cat. #: W4011; RRID:AB_430833 | WB (1:10,000) |
Antibody | anti-Mouse IgG-HRP (Goat monoclonal) | Promega | Cat. #: W4021; RRID:AB_430834 | WB (1:10,000) |
Antibody | anti-Rabbit IgG-Alexa fluor 488 (Goat monoclonal) | Invitrogen | Cat. #: A11034; RRID:AB_2576217 | IF (1:500) |
Antibody | anti-Chicken IgY-Alexa fluor 488 (Goat monoclonal) | Invitrogen | Cat. #: A11039; RRID:AB_2534096 | IF (1:500) |
Antibody | anti-Rabbit IgG-Alexa fluor Plus 647 (Goat monoclonal) | Invitrogen | Cat. #: A32733; RRID:AB_2633282 | IF (1:500) |
Antibody | anti-Guine pig IgG-Alexa fluor 405 (Goat monoclonal) | Abcam | Cat. #: ab175678; RRID:AB_2827755 | IF (1:500) |
Peptide, recombinant protein | PE-Cy7-Streptavidin | Biolegend | Cat. #: 405206 | FACS (3 µl per test) |
Peptide, recombinant protein | Alpha-bungarotoxin-Alexa fluor 555 | Invitrogen | Cat. #: B35451 | IF (1:1000) |
Peptide, recombinant protein | Alpha-bungarotoxin-Alexa fluor 488 | Invitrogen | Cat. #: B13422 | IF (1:1000) |
Peptide, recombinant protein | μ-conotoxin GIIIB | Alomone | Cat. #: C-270 | Electrophysiology (2.5 µM) |
Commercial assay or kit | Pierce BCA protein assay kits | Thermo Fisher Scientific | Cat. #: C-23225 | |
Software | ImageJ | ImageJ | RRID:SCR_003070 | |
Software | AccuCT | PerkinElmer | ||
Software | Microsoft Excel | Microsoft | RRID:SCR_016137 | |
Software | Leica Application Suite X | Leica | RRID:SCR_013673 | |
Software | GraphPad Prism | Graphpad | RRID:SCR_002798 | |
Software | pClamp | Molecular devices | RRID:SCR_011323 | |
Software | QIAGEN | QIAGEN | RRID:SCR_008539 | |
Other | Gill No. 3 formula hematoxylin | Sigma | Cat. #: GHS332 | Hematoxylin staining solution (1×) |
Other | Eosin Y solution | Sigma | Cat. #: HT110116 | Eosin staining solution (1×) |
Other | Toluidine blue | Sigma | Cat. #: 198161 | Toluidine blue staining (1%) |
Animals
Prrx1Cre (stock 005584), Smn1f7/+ (stock 006138), Rosa26 LSL-YFP/+ (stock 006148), Rosa26 LSL-tdTomato/+ (stock 007914), SMN2+/+, Smn1+/− (stock 005024 – Smn1 knockout and SMN2 homologous transgenic mouse) and Smn1+/−; SMN2+/+; SMNΔ7+/+ (stock 005025 005024 – Smn1 knockout and SMN2, SMNΔ7 homologous transgenic mouse) mice were acquired from The Jackson Laboratory (Bar Harbor, ME, USA). SMN2 0-copy Smn1ΔMPC mice (Prrx1Cre; Smn1f7/f7), SMN2 1-copy Smn1ΔMPC mice (Prrx1Cre; Smn1f7/f7; SMN2+/0 – SMN2 heterologous allele), and SMN2 2-copy Smn1ΔMPC mice (Prrx1Cre; Smn1f7/f7; SMN2+/+ – SMN2 homologous allele) were generated by crossing Prrx1Cre mice with Smn1f7/+ and Smn1+/−; SMN2+/+mice. To utilize FAPs transplantation, we generated Prrx1Cre; Rosa26 LSL-YFP/+ mice and Prrx1Cre; Rosa26 LSL-tdTomato/+ mice by breeding Prrx1Cre with Rosa26 LSL-YFP/+ and Rosa26 LSL-tdTomato/+ mice, respectively. To avoid deletion of the floxed allele by Prrx1Cre expression in female germline, we bred females without Prrx1Cre line to Prrx1Cre transgenic males. SMAΔ7 mutants (Smn1−/−; SMN2+/+; SMNΔ7+/+) were produced by mating Smn1+/−; SMN2+/+; SMNΔ7+/+ mice. Both male and female mice were used in the experiments, and no sex-specific differences were observed. Control littermates lacking Prrx1Cre were utilized for analysis. All mouse lines were housed under controlled conditions with specific pathogen free and handled according to the guidelines of the Seoul National University Institutional Animal Care and Use Committee (Protocol number: SNU-210313-1).
Micro-CT
The femurs from three groups – control, SMN2 2-copy Smn1ΔMPC, and SMN2 1-copy Smn1ΔMPC mice at postnatal day 14 (P14) – were isolated and cleaned of muscles and skin. Subsequently, the femurs were preserved in 4% paraformaldehyde (PFA) in phosphate-buffered saline (PBS) overnight at 4°C before micro-CT. The femurs were then imaged through a Quantum GX II micro-CT imaging system (PerkinElmer, Hopkinton, MA, USA). The X-ray source for scanning was set at 90 kV and 88 mA with a field of view of 10 mm (voxel size, 20 μm; scanning time, 14 min). The 3D imaging was viewed using the 3D Viewer software of the Quantum GX II. The size and volume of the femur bone were measured via AccuCT analysis software within the ossified diaphysis and metaphysis of the femur, excluding the epiphysis. BMD calibration was performed using a 4.5-mm BMD phantom and BMD measurements were taken at the center of the diaphysis.
Histology
Alcian blue and alizarin red staining was performed on control and SMN2 0-copy Smn1ΔMPC mice at E18.5 using a previously reported protocol (Ovchinnikov, 2009). For H&E and toluidine blue staining of the growth plate in the control and SMN2 1-copy Smn1ΔMPC mice at P14, the femurs were fixed in 4% PFA for 24 hr, rinsed in running tap water for 24 hr, and incubated with 10% ethylenediaminetetraacetic acid (EDTA) (pH 7.4) at 4°C with shaking for 2–3 days. Subsequently, the samples were rinsed in running tap water for 24 hr and then dehydrated through ethanol/xylene and embedded in paraffin. The embedded samples were then sectioned to a thickness of 5 µm, rehydrated, and stained with Gill No. 3 formula hematoxylin and eosin Y (H&E, Sigma-Aldrich, St. Louis, MO, USA) and toluidine blue (Sigma-Aldrich, St. Louis, MO, USA). Stained slides were analyzed using ×10 and ×20 objectives in the EVOS M7000 imaging system (Thermo Fisher Scientific, Waltham, MA, USA). The growth plate’s proliferative and hypertrophic zones were defined by their respective cell sizes.
Hindlimb FAPs isolation
Isolation of limb muscle FAPs was performed according to a previously reported protocol (Kim et al., 2020) with modifications. Limb muscles were dissected and mechanically dissociated in Dulbecco’s modified Eagle’s medium (DMEM, Hyclone) containing 10% horse serum (Hyclone, Logan, UT, USA), collagenase II (800 units/ml; Worthington, Lakewood, NJ, USA), and dispase (1.1 units/ml; Thermo Fisher Scientific, Waltham, MA, USA) at 37°C for 40 min. Digested suspensions were subsequently triturated by sterilized syringes with 20 G 1/2 needle (BD Biosciences, Franklin Lakes, NJ, USA) and washed with DMEM to harvest mononuclear cells. Mononuclear cells were stained with corresponding antibodies. All antibodies used in fluorescence-activated cell sorting (FACS) analysis are listed in Supplementary file 1. To exclude dead cells, 7AAD (Sigma-Aldrich; St. Louis, MO, USA) was used. Stained cells were analyzed and 7AAD−Lin−Vcam−Sca1+ (stem cell antigen 1; Ly6a) (FAPs) were isolated using FACS Aria III cell sorter (BD Biosciences) with four-way purity precision. For western blot, freshly isolated FAPs were cultured at 37°C in alpha-MEM (Hyclone) supplemented with Antibiotic–Antimycotic (anti–anti, Gibco) and 20% fetal bovine serum (FBS; Hyclone). For transplantation, YFP+ FAPs and tdTomato+ FAPs were isolated from postnatal day 10–21 Prrx1Cre; Rosa26 LSL-YFP/+ mice and Prrx1Cre; Rosa26 LSL-tdTomato/+ mice, respectively. Isotype control density plots were used as a reference for positive gating.
Chondrocytes isolation
Isolation of chondrocytes followed a modified protocol previously published (Jonason et al., 2015). Using blunt forceps, cartilage caps were removed from P21 mouse femoral heads and dissected into ~1 mm fragments in a Petri dish with 10× anti–anti in PBS. The cartilage fragments were washed twice with PBS and then incubated in 5 ml collagenase II solution (800 units/mL collagenase II in DMEM with anti–anti, sterilized by 0.2 µm filtration) in a 60 mm culture dish at 37°C in a 5% CO2 incubator overnight. Chondrocytes were released by pipetting the remaining cartilage fragments 10 times and then filtering them through a 70-μm cell strainer to a 50-ml conical tube. The cells were then washed twice with PBS and pelleted by centrifugation at 500 × g for 5 min. Subsequently, the cells were cultured overnight at 37°C in a 5% CO2 incubator, in complete culture medium (DMEM with 10% FBS and anti–anti) overnight at 37°C in a 5% CO2 incubator.
PCR reaction
To detect the presence of Smn1 exon 7 floxed and deleted allele, 20 mg of each tissue were dissolved in direct PCR buffer (VIAGEN) with proteinase K overnight at 65°C. After inactivation at 95°C for 30 min, PCR was performed using previously reported primer sets to verify the existence of Cre, Smn1f7, and Smn1Δ7 alleles (Frugier et al., 2000). Primers are listed in .
RNA extraction and measurement of mRNA expression
Total RNA was extracted from the brain, liver, spinal cord L4, tibialis anterior muscle, freshly isolated FAPs, and chondrocytes using a TRIzol Reagent (Life Technologies, Carlsbad, CA, USA) and analyzed by qRT-PCR. First-strand complementary DNA was synthesized from 1 μg of RNA using ReverTra Ace (Toyobo, Osaka, Japan) containing random oligomer according to the manufacturer’s instructions. qRT-PCR (QIAGEN) was performed with SYBR Green technology (SYBR Premix Ex Taq, QIAGEN) using specific primers against indicated genes. Relative mRNA levels were determined using the 2−ΔΔCt method and normalized to Gapdh (Figure 1H, Figure 1—figure supplement 1K). Primers are listed in Supplementary file 1.
Western blot
Cultured FAPs at passage 3 were homogenized in radioimmunoprecipitation assay (RIPA) buffer (50 mM Tris–HCl, pH 7.5, 0.5% sodium dodecyl sulfate, 20 µg/ml aprotinin, 20 µg/ml leupeptin, 10 µg/ml phenylmethylsulfonyl fluoride, 1 mM sodium orthovanadate, 10 mM sodium pyrophosphate, 10 mM sodium fluoride, and 1 mM dithiothreitol). Cell lysates were centrifuged at 13,000 rpm for 15 min. Supernatants were collected and subjected to immunoblot. BCA protein assay (Thermo Fisher Scientific) was used for estimating total protein concentrations. Normalized total proteins were analyzed by electrophoresis in 10% polyacrylamide gels and transferred to polyvinylidene fluoride (PVDF) membranes (Millipore, Billerica, MA, USA). Membranes were blocked in 5% skim milk (BD Biosciences) in tris-buffered saline (TBS) with 0.1% Tween-20 and incubated with primary antibodies overnight at 4°C. After incubation with the corresponding horseradish peroxidase (HRP)-conjugated secondary antibodies, the membranes were developed using a Fusion solo chemiluminescence imaging system (Vilber, Marne-la-Vallée, France). α-Tubulin was used as a loading control. Band intensities were quantified using ImageJ software. Antibodies used in this study are listed in Supplementary file 1. Primary and secondary antibodies were diluted 1:1000 and 1:10,000 with PBS containing 0.1% Tween-20 and 3% bovine serum albumin, respectively.
FAPs transplantation
FAPs transplantation was performed according to a previously reported protocol (Kim et al., 2020) with modifications. YFP+ or tdTomato+ FAPs (7AAD−Lin−Vcam−Sca1+) were isolated by FACS from the limb muscles of indicated mice. 1 × 105 FAPs were suspended in 0.1% gelatin (Sigma-Aldrich, St. Louis, MO, USA) in PBS and then transplanted into one side of the TA muscles of SMN2 1-copy Smn1ΔMPC mice. The contralateral muscle received an equivalent volume of 0.1% gelatin in PBS (Vehicle).
Electrophysiology
The EDL muscle was dissected from control and SMN2 1-copy Smn1ΔMPC mice, along with the peroneal nerve, and then pinned to a Sylgard-coated recording chamber. Intracellular recording was conducted in oxygenated Ringer’s solution, which comprised 138.8 mM NaCl, 4 mM KCl, 12 mM NaHCO3, 1 mM KH2PO4, 1 mM MgCl2, and 2 mM CaCl2 with a pH of 7.4. Action potential of the muscle was prevented by preparing the muscle in 2.5 μM μ-conotoxin GIIIB (Alomone, Jerusalem, Israel) for 10 min beforehand. The recording was performed in toxin-free Ringer’s solution. mEPPs were recorded from a junction, followed by recordings of eEPPs by stimulating the attached peroneal nerve. The eEPPs were elicited using evoked stimulation. Paired-pulse stimulation (10-ms interstimulus interval) was utilized to assess synaptic transmission. The data were obtained and analyzed with Axoclamp 900A and Clampfit version 10.7 software.
Transmission electron microscopy
NMJ TEM followed a modified protocol previously reported (Modla et al., 2010). Mouse EDL muscle was swiftly excised and fixed in 4% PFA dissolved in Sorensen’s phosphate buffer (0.1 M, pH 7.2), followed by washing in 0.1 M phosphate buffer. The EDL was then gradually infiltrated on a rotator at room temperature with sucrose: 0.1 M phosphate buffer solutions of 30% and 50%, for 1 hr each, followed by an overnight incubation in 70% sucrose. Excess sucrose was then eliminated using filter paper, and the muscle was embedded in an optimal cutting temperature compound (O.C.T.; Sakura Fineteck, Torrance, CA, USA), followed by being frozen in a cryostat (Leica, Wetzlar, Germany). 10-µm-thick longitudinal sections were washed in PBS and treated with Alexa fluor 555-conjugated α-bungarotoxin (1:500, Invitrogen) for an hour. Imaging was conducted with the EVOS M7000 imaging system, and we selected four to five NMJ-rich regions for processing with TEM. The sections were fixed with 2% glutaraldehyde and 2% PFA in 0.1 M cacodylate buffer (pH 7.2) for 2 hr at room temperature, with an additional overnight incubation at 4°C. After washing with 0.1 M cacodylate buffer they were post-fixed with 1% osmium tetraoxide in 0.1 M cacodylate buffer (pH 7.2) for 2 hr at 4°C. The sections were then stained en bloc with 0.5% uranyl acetate overnight, washed with distilled water, and dehydrated using serial ethanol and propylene oxide. The sections were embedded in epoxy resin (Embed-812, Electron Microscopy Sciences) and detached from the slides by dipping them in liquid nitrogen. Ultra-thin sections (70 nm) were prepared with a diamond knife on an ultramicrotome (ULTRACUT UC7, Leica) and mounted on 100 mesh copper grids. Sections were stained with 2% uranyl acetate for 10 min and lead citrate for 3 min, then observed using a transmission electron microscope (80 kV, JEM1010, JEOL or 120 kV, Talos L120C, FEI). Synaptic vesicle density was quantified within a distance of 500 nm from the presynaptic membrane.
Immunohistochemistry
For NMJ staining, freshly dissected TA muscles were fixed in 4% PFA for 30 min at room temperature. Subsequently, the muscles were cryoprotected in 30% sucrose overnight, embedded in O.C.T., snap-frozen in liquid nitrogen, and stored at −80°C prior to sectioning. Longitudinal 40-μm-thick sections were obtained from the embedded muscles using a cryostat. The sections were blocked for 2 hr at room temperature using 5% goat serum and 5% bovine serum albumin in PBS/0.4% Triton X-100. Then, the sections were incubated with primary antibodies in the blocking buffer for 2 days at 4°C. After washing the sections three times with PBS/0.4% Triton X-100, the sections were stained with secondary antibodies overnight at 4°C, and then incubated with Alexa fluor 488- or 555-conjugated α-bungarotoxin (1:500, Invitrogen) for 2 hr at room temperature (RT), washed three times with PBS/0.4% Triton X-100 and mounted in VECTASHIELD. Z-serial images were collected at ×40 with a Leica SP8 confocal laser scanning microscope. To analyze NMJ morphology, LasX software was used to obtain maximal projections. NF varicosity refers to the varicose NF end connected to the rest of the nerve terminal. To quantify NMJ size and synaptophysin coverage, the Btx area and synaptophysin area were measured by ImageJ analysis software.
For bone section staining, the 5-µm-thick bone sections were rehydrated and antigen retrieval was then performed in citrate buffer (10 mM citric acid, pH 6) at 95°C 20 min. The sections were blocked for 1 hr at room temperature using 5% goat serum and 5% bovine serum albumin in PBS/0.4% Triton X-100. Then, the sections were incubated with primary antibodies in the blocking buffer at 4°C overnight. After washing the sections three times with PBS/0.1% Triton X-100, the sections were stained with secondary antibodies for 1 hr at RT, washed and mounted. Imaging was conducted with the EVOS M7000 imaging system.
Statistical analysis
Sample size determination was based on anticipated variability and effect size that was observed in the investigator’s lab for similar experiments. For quantification, individual performing the counts were blinded to sample identity and randomized. All statistical analyses were performed using GraphPad Prism 9 (GraphPad Software). For comparison of significant differences in multiple groups for normally distributed data, statistical analysis was performed by one- or two-way analysis of variance (ANOVA) followed by Tukey’s pairwise comparison post hoc test. For non-normally distributed data, Brown–Forsythe and Welch ANOVA followed by Games–Howell multiple comparisons test was used. For the comparison of two groups, Student’s unpaired t-test assuming a two-tailed distribution with Welch’s correction was used. Unless otherwise noted, all error bars represent standard error of the mean. The number of biological replicates and statistical analyses for each experiment were indicated in the figure legends. Independent experiments were performed at least in triplicates. p < 0.05 was considered statistically significant at the 95% confidence level. *p < 0.05, **p < 0.01, ***p < 0.001, ****p < 0.0001.
Data availability
All data generated or analyzed during this study are included in the manuscript and supporting files; source data files have been provided for Figure 2.
References
-
Insights into muscle stem cell dynamics during postnatal developmentThe FEBS Journal 289:2710–2722.https://doi.org/10.1111/febs.15856
-
Role of insulin-like growth factors in embryonic and postnatal growthCell 75:73–82.
-
Increased IGF-1 in muscle modulates the phenotype of severe SMA miceHuman Molecular Genetics 20:1844–1853.https://doi.org/10.1093/hmg/ddr067
-
Spinal muscular atrophy: why do low levels of survival motor neuron protein make motor neurons sick?Nature Reviews. Neuroscience 10:597–609.https://doi.org/10.1038/nrn2670
-
Nusinersen: a treatment for spinal muscular atrophyThe Annals of Pharmacotherapy 53:61–69.https://doi.org/10.1177/1060028018789956
-
Emerging issues in AAV-mediated in vivo gene therapyMolecular Therapy - Methods & Clinical Development 8:87–104.https://doi.org/10.1016/j.omtm.2017.11.007
-
Growth patterns in children with spinal muscular atrophyOrphanet Journal of Rare Diseases 16:375.https://doi.org/10.1186/s13023-021-02015-9
-
SMN depleted mice offer a robust and rapid onset model of nonalcoholic fatty liver diseaseCellular and Molecular Gastroenterology and Hepatology 12:354–377.https://doi.org/10.1016/j.jcmgh.2021.01.019
-
Intravascular AAV9 preferentially targets neonatal neurons and adult astrocytesNature Biotechnology 27:59–65.https://doi.org/10.1038/nbt.1515
-
Nuclear targeting defect of SMN lacking the C-terminus in a mouse model of spinal muscular atrophyHuman Molecular Genetics 9:849–858.https://doi.org/10.1093/hmg/9.5.849
-
Growth plate chondrocytes: skeletal development, growth and beyondInternational Journal of Molecular Sciences 20:6009.https://doi.org/10.3390/ijms20236009
-
Comparison between mechanical stress and bone mineral density in the femur after total hip arthroplasty by using subject-specific finite element analysesComputer Methods in Biomechanics and Biomedical Engineering 18:1056–1065.https://doi.org/10.1080/10255842.2013.869320
-
BookPrimary murine growth plate and Articular Chondrocyte isolation and cell cultureIn: Westendorf JJ, van Wijnen AJ, editors. Osteoporosis and Osteoarthritis. Humana Press. pp. 11–18.https://doi.org/10.1007/978-1-4939-1619-1
-
Genetic regulation of the growth plateFrontiers in Endocrinology 2:113.https://doi.org/10.3389/fendo.2011.00113
-
Reduced SMN protein impairs maturation of the neuromuscular junctions in mouse models of spinal muscular atrophyHuman Molecular Genetics 17:2552–2569.https://doi.org/10.1093/hmg/ddn156
-
Requirement of enhanced Survival Motoneuron protein imposed during neuromuscular junction maturationThe Journal of Clinical Investigation 124:785–800.https://doi.org/10.1172/JCI72017
-
Low bone mineral density in spinal muscular atrophyJournal of Clinical Neuromuscular Disease 10:11–17.https://doi.org/10.1097/CND.0b013e318183e0fa
-
Muscle-specific SMN reduction reveals motor neuron-independent disease in spinal muscular atrophy modelsThe Journal of Clinical Investigation 130:1271–1287.https://doi.org/10.1172/JCI131989
-
Bone health and growth in spinal muscular atrophy type 2 and 3Journal of Istanbul Faculty of Medicine / İstanbul Tıp Fakültesi Dergisi 01:22–28.https://doi.org/10.26650/IUITFD.884235
-
Survival motor neuron protein in motor neurons determines synaptic integrity in spinal muscular atrophyThe Journal of Neuroscience 32:8703–8715.https://doi.org/10.1523/JNEUROSCI.0204-12.2012
-
Spinal muscular atrophy - insights and challenges in the treatment eraNature Reviews. Neurology 16:706–715.https://doi.org/10.1038/s41582-020-00413-4
-
Spinal muscular atrophyNature Reviews. Disease Primers 8:.https://doi.org/10.1038/s41572-022-00380-8
-
Identification of neuromuscular junctions by correlative confocal and transmission electron microscopyJournal of Neuroscience Methods 191:158–165.https://doi.org/10.1016/j.jneumeth.2010.06.015
-
Non-myogenic contribution to muscle development and homeostasis: the role of connective tissuesFrontiers in Cell and Developmental Biology 5:22.https://doi.org/10.3389/fcell.2017.00022
-
Alcian blue/alizarin red staining of cartilage and bone in mouseCold Spring Harbor Protocols 2009:pdb.prot5170.https://doi.org/10.1101/pdb.prot5170
-
CNS-targeted gene therapy improves survival and motor function in a mouse model of spinal muscular atrophyThe Journal of Clinical Investigation 120:1253–1264.https://doi.org/10.1172/JCI41615
-
Adeno-associated virus vector genomes persist as episomal chromatin in primate muscleJournal of Virology 82:7875–7885.https://doi.org/10.1128/JVI.00649-08
-
The actions of IGF-1 in the growth plate and its role in postnatal bone elongationCurrent Osteoporosis Reports 18:210–227.https://doi.org/10.1007/s11914-020-00570-x
-
Multiple roles of integrin-α3 at the neuromuscular junctionJournal of Cell Science 130:1772–1784.https://doi.org/10.1242/jcs.201103
-
Pathological impact of SMN2 mis-splicing in adult SMA miceEMBO Molecular Medicine 5:1586–1601.https://doi.org/10.1002/emmm.201302567
-
The developmental basis of mesenchymal stem/stromal cells (MSCs)BMC Developmental Biology 15:44.https://doi.org/10.1186/s12861-015-0094-5
-
Pubertal growth and epiphyseal fusionAnnals of Pediatric Endocrinology & Metabolism 20:8–12.https://doi.org/10.6065/apem.2015.20.1.8
-
Mesenchymal Bmp3b expression maintains skeletal muscle integrity and decreases in age-related sarcopeniaThe Journal of Clinical Investigation 131:e139617.https://doi.org/10.1172/JCI139617
-
Systemic delivery of scAAV9 expressing SMN prolongs survival in a model of spinal muscular atrophyScience Translational Medicine 2:35ra42.https://doi.org/10.1126/scitranslmed.3000830
-
IGF-1R signaling in chondrocytes modulates growth plate development by interacting with the PTHrP/Ihh pathwayJournal of Bone and Mineral Research 26:1437–1446.https://doi.org/10.1002/jbmr.359
-
Circulating levels of IGF-1 directly regulate bone growth and densityThe Journal of Clinical Investigation 110:771–781.https://doi.org/10.1172/JCI15463
-
Serum IGF1 and IGFBP3 levels in SMA patientsNeuromuscular Disorders 26:S105.https://doi.org/10.1016/j.nmd.2016.06.075
Article and author information
Author details
Funding
National Research Foundation of Korea (NRF-2022R1A2C3007621)
- Young-Yun Kong
National Research Foundation of Korea (NRF-2020R1A5A1018081)
- Young-Yun Kong
National Research Foundation of Korea (NRF-2020R1A2C3011464)
- Se-Young Choi
The funders had no role in study design, data collection, and interpretation, or the decision to submit the work for publication.
Acknowledgements
We express our gratitude to the Kong and Choi laboratory members for their valuable feedback during the project. This work was supported by the National Research Foundation of Korea (NRF-2022R1A2C3007621, NRF-2020R1A5A1018081, and NRF-2020R1A2C3011464).
Ethics
The care and treatment of animals in this study were approved by the Institutional Animal Care and Use Committee (IACUC) protocols (SNU-210313-1) of Seoul National University.
Version history
- Sent for peer review: September 21, 2023
- Preprint posted: October 10, 2023 (view preprint)
- Preprint posted: November 7, 2023 (view preprint)
- Preprint posted: January 23, 2024 (view preprint)
- Version of Record published: February 6, 2024 (version 1)
Cite all versions
You can cite all versions using the DOI https://doi.org/10.7554/eLife.92731. This DOI represents all versions, and will always resolve to the latest one.
Copyright
© 2023, Hann, Kim et al.
This article is distributed under the terms of the Creative Commons Attribution License, which permits unrestricted use and redistribution provided that the original author and source are credited.
Metrics
-
- 727
- views
-
- 106
- downloads
-
- 0
- citations
Views, downloads and citations are aggregated across all versions of this paper published by eLife.
Download links
Downloads (link to download the article as PDF)
Open citations (links to open the citations from this article in various online reference manager services)
Cite this article (links to download the citations from this article in formats compatible with various reference manager tools)
Further reading
-
- Medicine
Caesarean section scar diverticulum (CSD) is a significant cause of infertility among women who have previously had a Caesarean section, primarily due to persistent inflammatory exudation associated with this condition. Even though abnormal bacterial composition is identified as a critical factor leading to this chronic inflammation, clinical data suggest that a long-term cure is often unattainable with antibiotic treatment alone. In our study, we employed metagenomic analysis and mass spectrometry techniques to investigate the fungal composition in CSD and its interaction with bacteria. We discovered that local fungal abnormalities in CSD can disrupt the stability of the bacterial population and the entire microbial community by altering bacterial abundance via specific metabolites. For instance, Lachnellula suecica reduces the abundance of several Lactobacillus spp., such as Lactobacillus jensenii, by diminishing the production of metabolites like Goyaglycoside A and Janthitrem E. Concurrently, Clavispora lusitaniae and Ophiocordyceps australis can synergistically impact the abundance of Lactobacillus spp. by modulating metabolite abundance. Our findings underscore that abnormal fungal composition and activity are key drivers of local bacterial dysbiosis in CSD.
-
- Medicine
- Neuroscience
Gait is impaired in musculoskeletal conditions, such as knee arthropathy. Gait analysis is used in clinical practice to inform diagnosis and to monitor disease progression or intervention response. However, clinical gait analysis relies on subjective visual observation of walking, as objective gait analysis has not been possible within clinical settings due to the expensive equipment, large-scale facilities, and highly trained staff required. Relatively low-cost wearable digital insoles may offer a solution to these challenges. In this work, we demonstrate how a digital insole measuring osteoarthritis-specific gait signatures yields similar results to the clinical gait-lab standard. To achieve this, we constructed a machine learning model, trained on force plate data collected in participants with knee arthropathy and controls. This model was highly predictive of force plate data from a validation set (area under the receiver operating characteristics curve [auROC] = 0.86; area under the precision-recall curve [auPR] = 0.90) and of a separate, independent digital insole dataset containing control and knee osteoarthritis subjects (auROC = 0.83; auPR = 0.86). After showing that digital insole derived gait characteristics are comparable to traditional gait measurements, we next showed that a single stride of raw sensor time series data could be accurately assigned to each subject, highlighting that individuals using digital insoles can be identified by their gait characteristics. This work provides a framework for a promising alternative to traditional clinical gait analysis methods, adds to the growing body of knowledge regarding wearable technology analytical pipelines, and supports clinical development of at-home gait assessments, with the potential to improve the ease, frequency, and depth of patient monitoring.